Photosynthetic physiology and stress-resistant biochemical properties reveal the invasive photo-adaptation strategy of marine green alga Codium fragile
-
Abstract: Biological invasions have become recognized as one of the greatest threats to ecosystems. Codium, a genus of invasive green algae, has frequent global outbreaks and damages local marine ecosystems. It is now generally accepted that light is one of the main factors affecting the luxuriant growth of macroalgae such as Codium. In this study, to investigate the invasive photo-adaptation strategy of Codium fragile, the photo-adaptation characteristics of C. fragile and C. cylindricum from the Nan’ ao Island of China were compared and explored. The effect of light intensity on the photosynthetic properties of the two species was investigated: the maximum quantum yield of photosystem II (Fv/Fm) of C. fragile was significantly higher at low light intensity. At a light intensity of 90 μmol/(m2·s), maximum relative electron transport rate (rETRmax) of the thalli was maximum, and the minimum saturating irradiance (Ek) was significantly increased. The photosynthetic rate (α value) of thalli was highest at a light intensity of 30 μmol/(m2·s). The photochemical quenching (qP) was enhanced but non-photochemical quenching (NPQ) was reduced at high light intensities. As for C. cylindricum, the optimal photochemical efficiency of the thalli at low light intensity was higher. High light intensity significantly reduced the rETR of the thalli. At low light intensity, α was significantly higher, Ek was significantly lower, and NPQ was also significantly decreased. The response relationship between light acclimation and antioxidant capacity of the thalli of two species of Codium was investigated: there was no significant effect of light intensity variation on the total antioxidant capacity of C. fragile. In the case of C. cylindricum, the degree of membrane lipid peroxidation was significantly increased at low light intensity, and its antioxidant capacity was significantly reduced when the light intensity was too high or too low. It can be hypothesized that the self-protection ability of C. fragile may be stronger than that of C. cylindricum under low and high light intensities, which is closely related to the strong invasiveness of C. fragile.
-
Key words:
- Codium fragile /
- invasive algae /
- photo-adaptation /
- photosynthesis
-
1. Introduction
In recent years, biological invasions have gradually become the greatest threat to ecosystems. Codium is a group of large, multinucleate, tubular green algae with an intertidal or subtidal distribution in the tropics and subtropics (Silva, 1955). Some species of this genus are recognized as one of the greatest threats to shallow water ecosystems due to their proliferation as invasive seaweeds (Vitousek et al., 1957), such as Codium fragile, which encroach on the habitat of other organisms (Drouin et al., 2012).
On the other hand, Codium is also an important genus of seaweed resources. The extract of C. tomentosum can be used as the raw material of cosmetics (Wang et al., 2015; Leandro et al., 2020). Extract of C. cylindricum can act as an anticoagulant and antioxidant (Matsubara et al., 2001; Yan et al., 2021). Codium fragile has the effect of expelling parasites from the human body, and its extract also has antitumor, antibacterial, and anticoagulant effects (Yin et al., 2007; Ye et al., 2010; Wei and Zheng, 2021). Therefore, research on the physiological characteristics of Codium is important for both biological invasion control and seaweed resource development.
How Codium can invade successfully has attracted the attention of several scholars. Contrary to popular belief, some species of this genus can be attached to soft substrates rather than only to hard substrates (Williams, 2007). In addition, their broad physiological tolerance and high growth rate in summer and early autumn are also conducive to their widespread expansion (Yang et al., 1997; Malinowski and Ramus, 1973; Hanisak, 1979; Benson et al., 1983; Hanisak and Harlin, 1978). Hanisak (1979) systematically studied the effects of temperature, light, salinity, and nitrogen source on the growth of C. fragile in combination with field experiments and indoor culture, and found that the growth pattern of C. fragile in the field was mainly affected by temperature and light intensity. Marques et al. (2022) considered light availability as a major parameter affecting the growth of macroalgae.
However, there are few studies on the physiological and biochemical responses of Codium species to the above environmental factors. Some scholars believe that light is the main factor affecting the growth of macroalgae such as Codium (Marques et al., 2022; Magnusson et al., 2015), and light also regulates the effects of other environmental stressors on the photosynthetic performance of algae (Gao et al., 2016, 2018). To investigate the relationship between the invasiveness of Codium species and their photo-adaptation, two species C. fragile and C. cylindricum were selected as experimental samples for a comparative study. Codium fragile is native to Japan and has now invaded several regions around the world, including the northwest Atlantic coast, the U.S. State of Florida, southern New England, the Mediterranean Sea, Australia, New Zealand, and Chile, mainly in the intertidal zone (Silva, 1955; Israel et al., 2010; Trowbridge, 1995; Bird et al., 1993; Carlton and Scanlon, 1985; Chapman, 1998; Neill et al., 2006; Churchill and Moeller, 1972; Pérez-Cirera et al., 1989; Lapointe et al., 2005; Dromgoole, 1975; Ding et al., 2022). According to the data from AlgaeBase, C. cylindricum, which was also first recorded in Japan, is now mainly distributed in Japan, Korea, the South China Sea, and coastal areas of Southeast Asia, growing mostly in the lower intertidal to subtidal areas. In this study, the chlorophyll fluorescence analysis technique was used to indirectly measure the changes in their photosynthesis and to examine their photosynthetic physiological responses to different light intensities. The pigment content, malondialdehyde content, peroxidase activity, and total antioxidant capacity (T-AOC) were also measured for the investigation of their physiological and biochemical responses to different light intensities. The invasive photo-adaptation strategies of C. fragile were inferred and generalized based on comparing the differences among photosynthetic physiological, physiological, and biochemical responses of the two species of Codium to different light intensities.
2. Materials and methods
2.1 Materials preparation
The mature spongiform thalli of C. fragile and C. cylindrical were collected from the Shen’ao Bay, Nan’ao Island, China in March 2011. The epiphytes and protozoa on the surface of the specimen were removed with paintbrushes, and the specimens were rinsed several times with sterile seawater and checked under the dissecting microscope (Olympus LG-PS 2, Japan). The thalli were divided into several parts and were cultured in
1000 mL clear plastic bottles containing sterile natural seawater (salinity of 30).2.2 Laboratory culture
The thalli were cultured in 20℃ climate-controlled culture chambers (GXZ-380 B, China) and were illuminated by daylight-type, white fluorescent tubes, photoperiod 12:12 (L:D). Five tiers of illuminance were designed, which were 10, 30, 60, 90, and 120 μmol/(m2∙s). To reduce the effects of pollution, the medium was replaced every day.
2.3 Rapid light curve (RLC) analysis
The optimal photochemical efficiency of photosynthesis (Fv/Fm) and rapid light curve (RLC) were measured with an imaging-PAM fluorometer (PAM-Water-ED, Walz, Germany). The samples were exposed to successive light intensity (calculated by photons) gradients (PAR 0, 21, 56, 55, 111, 186, 281, 336, 396, 461, 531 μmol/(m2∙s)) for 10 s each step. The relative electron transfer rate (rETR) and the effective quantum yield of PSII were calculated simultaneously at each light intensity according to the following equation (Schreiber, 2004):
$$ \left.\begin{array}{l} \mathrm{rETR=} {Y} \mathrm{(II)}\times PAR\times 0.84\times 0.5\\ {Y} \mathrm{(II)} =({F}'_{ \mathrm{m}} - {F} _{ \mathrm{s}} )/ {F}^\prime _{ \mathrm{m}} \end{array}\right\} $$ (1) where Y(II) is the efficient quantum yield of photosystem II, F'm is the light-adapted maximum fluorescence and Fs is the light-adapted steady-state fluorescence. Rapid light curves were fitted according to the following equation (Platt et al., 1980):
$$ {P} = {P}_{ \mathrm{m}} ({1-e}^{ -{\alpha } \times \mathrm{PAR/} {P}_\mathrm{m}} ) {e}^{ - {\beta } \times \mathrm{PAR/} {P}_\mathrm{m}} , $$ (2) where P is the photosynthetic rate or relative electron transfer rate, Pm is the maximum relative electron transfer rate (rETRmax), α is the photosynthetic rate in the light-limited region of RLC, and β is the photoinhibition parameter.
In addition, the minimum saturating irradiance (Ek) was calculated according to the following equation (Bouman et al., 2018):
$$ \mathit{E} _{ \mathrm{k}} \mathrm= \mathit{P} _{ \mathrm{m}} \mathrm{/} \mathit{\alpha } \mathrm{.} $$ (3) 2.4 Chlorophyll fluorescence quenching analysis
The photochemical quenching (qP) and non-photochemical quenching (NPQ) coefficients of chlorophyll fluorescence are calculated based on the internal function of Imaging-PAM with the following equation (Guidi and Degl’Innocenti, 2012):
$$ \left. \begin{array}{l}\mathrm{qP} =( {F}'_{\mathrm{m}} - {F} _{ \mathrm{t}} )/ ( {F}' _{ \mathrm{m}} - {F} _{ \mathrm{0}} )\\ \mathrm{NPQ} =( {F} _{ \mathrm{m}} - {F}'_{ \mathrm{m}} / {F}'_{ \mathrm{m}} \end{array}\right\} $$ (4) 2.5 Photosynthetic pigments measurement
After 7 d of culture, 0.1 g of thalli (FW) was weighed and extracted with 5 mL of 100% methanol. The extract was stored in a refrigerator at −4℃ for 24 h in the dark. Whereafter, the extract was centrifuged at 5 000× g and 4℃ for 10 min. The absorbance of the extract was measured with an ultraviolet-visible spectrophotometer (Shimadzu, UV-2450, Japan) and the control group was methanol. The content of chlorophyll a (Chl a) and carotenoids (Car) was calculated according to the following equation (Porra, 2002; Parsons and Strickland, 1963):
$$ \left.\begin{array}{l} {[\mathrm{Chl}\ a]} =16.29 \times A_{665}-8.54 \times A_{652} \\ {[\mathrm{Car}]} =7.6 \times\left(A_{480}-1.49 \times A_{510}\right) \end{array}\right\} $$ (5) 2.6 Malondialdehyde measurement
A total of 0.2 g of thalli (FW) that was cultured for 7 d was weighed and cut into pieces, and 2 mL of 10% TCA and a small amount of quartz sand were mixed with the pieces of thalli. The mixture was ground until homogenized. Then 2 mL of 10% TCA was added for further grinding, and the homogenate was centrifuged at 4 000 r/min for 10 min. The supernatant was the sample extract. A total of 2 mL of supernatant was aspirated (2 mL of distilled water for the control) and mixed with 2 mL of 0.6% TBA solution. The test tubes containing the mixture were boiled for 10 min (timed from the appearance of small bubbles in the solution in the test tubes). The tubes were then cooled, and centrifuged at 3 000 r/min for 15 min. The supernatant was collected and the absorbance values at 532 nm, 600 nm, and 450 nm were measured (the control group was used as a blank control). The concentration (cMDA, µmol/L) of malondialdehyde (MDA) was calculated according to the following equation:
$$ {c} _{ \mathrm{MDA}} = 6.45 ( {A}_{{532}} - {A}_{600} )-0.56 {A} _{450} $$ (6) where A450, A532, and A600 denote the absorbance values at 450 nm, 532 nm, and 600 nm, respectively. The content of MDA (CMDA, μmol/g) was then calculated using the following equation:
$$ \mathit{C} _{ \mathrm{MDA}} \mathrm{=(} \mathit{c} _{ \mathrm{MDA}} \mathit{V} _{ \mathrm{T}} \mathit{V} _{ \mathrm{1}} \mathrm{)/(1000} \mathit{V} _{ \mathrm{2}} \mathit{W} \mathrm{),} $$ (7) where VT is the total volume of the extract, Vl is the total volume of the extract and TBA solution reacted, V2 is the volume of the extract reacted with TBA, and W is the mass of the fresh sample.
2.7 Peroxidase activity measurement
After 7 d of culture, 0.2 g of thalli was weighed and mixed with 5 mL of 20 mmol/L KH2PO4. The mixture was ground into a homogenate in a mortar, centrifuged at 4 000 r/min for 15 min, and the supernatant was collected and stored in a cold place. The residue was extracted once more with 5 mL of KH2PO4 solution, and the supernatant of both times was combined. Take 2 colorimetric cups with an optical diameter of 1 cm. Add 3 mL of reaction mixture and 1 mL of KH2PO4 to one of the cups as a zero control, and add 3 mL of reaction mixture and l mL of enzyme solution to the other cup. The stopwatch was then turned on immediately and the OD value was measured at 470 nm in the spectrophotometer for 5 min, with readings taken at 1 min intervals. A curve was then made of the value of the change in OD with reaction time, and the slope of the initial linear portion of the curve was calculated as the value of the change in OD, i.e., ∆A470, expressed in [min·mg protein (or fresh weight g)].
2.8 Total antioxidant capacity (T-AOC) measurement
The total antioxidant capacity (T-AOC) of thalli was determined according to the instructions of the total antioxidant capacity assay kit of Nanjing Jiancheng Bioengineering Institute. The sample was weighed accurately and added 5 times the volume of phosphate-buffered saline (PBS) pH 7.0 at the ratio of weight (g): volume (mL) = 1:5, and the homogenate was prepared in an ice-water bath, centrifuged at 2 500 r/min for 10 min, and the supernatant was collected for measurement. The operation is according to Table 1.
Table 1. Operational procedure for the determination of T-AOCMeasurement tube Control tube Reagent 1/mL 1 1 Sample to be tested/mL a* Reagent 2/mL 2 2 Reagent 3/mL 0.5 0.5 Mix thoroughly with a vortex mixer, 37℃ water bath for 30 min Reagent 4/mL 0.2 0.2 Sample to be tested/mL a* Reagent 5/mL 0.2 0.2 Note: The reference sampling volume for 10% tissue homogenate is 0.2 mL. Mix the reaction solution well and leave at 25−37℃ for 10 min. Set the spectrophotometer at 520 nm, 1 cm optical diameter, zero with double-distilled water, and measure the absorbance value of each tube.
The total antioxidant capacity was defined as one unit of total antioxidant capacity for every 0.01 increase in the absorbance (OD) of the reaction system per minute per gram of fresh weight thalli at 37℃. The total antioxidant capacity (T-AOC) was calculated according to the following equation:
$$ \mathrm{T-AOC}=\left[\left(\mathrm{OD}_{\mathrm{m}}-\mathrm{OD}_{\mathrm{c}}\right) / 0.01\right] \div 30 \times\left(V_{\mathrm{f}} / V_{\mathrm{s}}\right) \div \mathrm{FW} $$ (8) Where ODm is the OD of the sample, ODc is the OD of control, Vr is the total volume of the reaction solution, Vs is the volume of the sample and FW is the fresh weight (g) of the sample.
2.9 Statistical analysis
The rapid light curves were linearly fitted using SPSS 20. The data were processed using GraphPad Prism 5.0 and Excel. Significant differences between treatments were analyzed by one-way ANOVA or T-test, and the significance level was set at P<0.05.
3. Results
3.1 Effect of light on photosynthetic physiology of C. fragile and C. cylindricum
In the light intensity range of 10−120 μmol/(m2∙s), the optimal photochemical efficiency of photosynthesis (Fv/Fm) of C. fragile showed a decreasing trend, and the low light intensity group (10−30 μmol/(m2∙s)) had higher photosynthetic efficiency than the high light intensity group (60−120 μmol/(m2∙s)), and there was a significant difference. Whereas, the rapid light curves (RLCs) showed that the relative electron transfer rate (rETR) of the thalli was the lowest at low light intensities (10 μmol/(m2∙s)). The convexity of the rapid light curve (RLC) was the highest at a light intensity of 90 μmol/(m2∙s), and there was no sign of a decrease in the rETR with the increase in light intensity. The rETRmax showed a trend of rising and then decreasing. It reached a maximum at a light intensity of 90 μmol/(m2∙s). The initial slope of the rapid light curve (α) tended to rise and then fall, and the α value was maximum at a light intensity of 30 μmol/(m2∙s) and was significantly different from that of the high light intensity group. The minimum saturating irradiance (Ek) showed a rising and then decreasing trend, and increased significantly at 90 μmol/(m2∙s) (Figs 1−3).
Figure 1. The Fv/Fm of two Codium species cultured at different light intensities. a. The Fv/Fm of C. fragile cultured at different light intensities, and b. the Fv/Fm of C. cylindricum cultured at different light intensities. Different letters (a, b, c, and d) represent significant differences among groups.Figure 3. The rETRmax, photosynthetic rate in the light-limited region of RLC (α), and Ek of two Codium species cultured at different light intensities. The data were obtained from RLCs. a. The comparison of rETRmax of C. fragile cultured at different light intensities, b. the comparison of rETRmax of C. cylindricum cultured at different light intensities, c. the comparison of α of C. fragile cultured at different light intensities, d. the comparison of α of C. cylindricum cultured at different light intensities, e. the comparison of Ek of C. fragile cultured at different light intensities, and f. the comparison of Ek of C. cylindricum cultured at different light intensities. Different letters (a, b, and c) represent significant differences among groups.The photochemical quenching curve (qP) was significantly lower in the lower light intensity range (10−60 μmol/(m2∙s)) than in the higher light intensity range (90−120 μmol/(m2∙s)), and the non-photochemical quenching curve (NPQ) of C. fragile was significantly higher at a light intensity of 120 μmol/(m2∙s) than the curves of other light groups, and the lowest NPQ was observed at a light intensity of 90 μmol/(m2∙s) (Figs 4 and 5).
For C. cylindricum, the Fv/Fm tended to decrease in the light range of 10−120 μmol/(m2∙s), and the maximal photochemical efficiency was higher in the low light intensity group (10−30 μmol/(m2∙s)) than in the high light intensity group (90−120 μmol/(m2∙s)), with a significant difference. The RLCs showed that the rETR of the thalli was lowest at high light intensities (120 μmol/(m2∙s)), and the convexity of the RLC was higher than the other groups at a light intensity of 30 μmol/(m2∙s). The rETRmax tended to increase and then decrease and reached a maximum at a light intensity of 30 μmol/(m2∙s). The α tended to decrease gradually, and the α values of the low light intensity group (10−30 μmol/(m2∙s)) were significantly different from those of the high light intensity group (90−120 μmol/(m2∙s)). The Ek showed a gradual increase, and the Ek values in the low light intensity group (10−30 μmol/(m2∙s)) were significantly different from those in the high light intensity group (90−120 μmol/(m2∙s)) (Figs 1−3).
The qP was lower in the lower light intensity range (10−60 μmol/(m2∙s)) than in the higher light intensity range (90−120 μmol/(m2∙s)). The NPQ of C. cylindricum were significantly higher in the low light intensity group (10−30 μmol/(m2∙s)) than in the high light intensity group (90−120 μmol/(m2∙s)) (Figs 4 and 5).
3.2 Physiological and biochemical responses of spongiform thalli of C. fragile and C. cylindricum to different light intensities
Codium fragile was able to grow in the light intensity range of 10−120 μmol/(m2∙s) with no significant difference in chlorophyll content. Carotenoids showed an increasing trend in the light intensity range of 30−120 μmol/(m2∙s) (Fig. 6). The concentration of malondialdehyde (MDA) and peroxidase (POD) activity tended to increase with light enhancement, but there was no significant difference (Figs 7 and 8). The total antioxidant capacity (T-AOC) of thalli tended to increase and then decrease with the increase of light intensity, and the strongest total antioxidant capacity of thalli was found in the range of 60−90 μmol/(m2∙s) (Fig. 8).
Figure 8. The comparison of POD activity (a, letters a represent no significant differences among groups (p>0.05, ANOVA, followed by Tukey's multiple comparison test)) and T-AOC (b, different letters (a and b) represent significant differences among groups (p<0.05, ANOVA, followed by Tukey's multiple comparison test)) of two Codium species under different light intensities.For C. cylindricum, it was able to grow in the light intensity range of 10−120 μmol/(m2∙s) with no significant difference in chlorophyll and carotenoids content (Fig. 6). In the range of 10−120 μmol/(m2∙s), the malondialdehyde (MDA) content of thalli showed a decreasing trend, and the highest malondialdehyde content of thalli was found at 10 μmol/(m2∙s) and significantly different from the high luminosity group (90 μmol/(m2∙s)) (Fig. 7). Peroxidase (POD) activity tended to increase with the enhancement of light (Fig. 8). And the total antioxidant capacity (T-AOC) increased first and then decreased with the enhancement of light. The total antioxidant capacity was strongest at the light intensity of 60 μmol/(m2∙s), which was significantly different from the low-light group (10 μmol/(m2∙s)) and high-light group (90−120 μmol/(m2∙s)), respectively (Fig. 8).
4. Discussion
The growth, reproduction, and chemical composition of seaweeds are influenced by the interaction of temperature, nutrients, and light (Marques et al., 2021). Therefore, the study of the combined effects of these factors on Codium is important for both invasion control and culture. Fv/Fm is the maximum photochemical quantum yield of PSII, reflecting the efficiency of light energy conversion within the PSII reaction center. The value varied little under non-stress conditions and was not affected by species and growth conditions, and it decreased significantly under stress conditions (Xu et al., 1992). In the light intensity range of 10−120 μmol/(m2∙s) in this paper, the Fv/Fm of both C. fragile and C. cylindricum showed a decreasing trend, and the low light intensity group (10−30 μmol/(m2∙s)) had higher photosynthetic efficiency than the high light intensity group (60−120 μmol/(m2∙s)), with significant differences. The decrease in Fv/Fm indicates that PSII was damaged by light-intensity stress, which reduced the primary light energy transformation efficiency of PSII, damaged the PSII active center, and inhibited the primary reaction of photosynthesis (Hui et al., 2004). This may be because the transfer of photosynthetic electrons from the PSII reaction center to QA and other pools is affected by excessive light intensity, and the PSII active center is damaged (Yang et al., 2004). Similarly, high light intensity also affects the ability of algae to respond to light (Ma et al., 2006), as the rETRmax of thalli is significantly reduced at high light intensities (120 μmol/(m2∙s)).
When plants capture more light energy than they need for photosynthesis, the excess energy is dissipated through NPQ to protect photosynthetic structures from damage (Maxwell and Johnson, 2000). Goss et al. (1998) suggested that NPQ reflects the strength of the radiationless energy dissipation pathway of the xanthophyll cycle. The xanthophyll cycle promotes the conformational change of photosystem II light-harvesting complexes (LHCII) from the “light-funnel” state to the “energy-consuming” state (Ruban et al., 1994). The results of this study showed that high light intensity caused an increase in the NPQ curve of C. fragile, indicating that as the photosynthetic capacity of the thalli decreased, more energy was consumed in the form of thermal energy. The excess light energy absorbed by the thalli can also be consumed through the molecular oxygen uptake pathway, which directly results in a large production of various reactive oxygen species (ROS) (Ciompi et al., 1996). It further causes damage to the cell membrane system and peroxidation of membrane lipids, and even leads to cell death (Van Breusegem et al., 2001). In contrast, the NPQ curve of C. cylindricum decreased at a high light intensity, and thus the excess light energy was likely to be consumed by the molecular oxygen uptake pathway. It is probably because of this that the total antioxidant capacity of the thalli decreased significantly under high light intensity.
In the light range of 10−120 μmol/(m2∙s), the MDA of C. fragile increased with the increase of light intensity. The MDA content was higher at high light intensities (90−120 μmol/(m2∙s)) but was not significantly different from the low light intensity group. In contrast, C. cylindricum showed essentially the opposite trend in the same light intensity range, with its MDA content decreasing with increasing light intensity. This implies that the degree of membrane lipid peroxidation in C. fragile increases with increasing light intensity, while the opposite is essentially true for C. cylindricum. Carotenoids protect plant cells and tissues from photo-oxidative damage by scavenging reactive oxygen species through physical and chemical reactions (Maoka, 2020). The carotenoids of C. fragile showed an increasing trend in the light range of 30−120 μmol/(m2∙s). In contrast, no significant differences were found in the carotenoid content of C. cylindricum in the light range of 10−120 μmol/(m2∙s). Therefore, it can be speculated that C. fragile can resist the oxidative damage to thalli cells by strong light through the accumulation of carotenoids under a high light intensity environment, and mitigate the degree of membrane lipid peroxidation to a certain extent.
Algae have antioxidant systems that resist external damage, including enzymes and small-molecule antioxidant substances (Zubia et al., 2007). POD is the main enzyme that decomposes H2O2 into water (H2O) and is a component of the antioxidant system and a component of the protective enzyme system (Velikova et al., 2000). The level of POD activity affects the decomposition of hydrogen peroxide and other peroxides. Peroxides in plants are toxic substances, and low concentrations of peroxides are also capable of blocking photosynthesis (Du et al., 2001). In the light intensity range of 10−120 μmol/(m2∙s), the POD activity of both C. fragile and C. cylindricum showed an increasing trend with light enhancement. At lower light intensities (10−30 μmol/(m2∙s)), the POD activity of C. fragile was significantly higher than that of C. cylindricum. Moreover, low light intensity (10 μmol/(m2∙s)) and high light intensity (120 μmol/(m2∙s)) had no significant effect on the T-AOC of C. fragile, but the T-AOC of C. cylindricum was significantly reduced. We speculate that the self-protective ability of C. fragile may be stronger than that of C. cylindricum at low and high light intensities and that the high peroxidase activity and stable total antioxidant capacity of C. fragile at high light intensities may mitigate the effects of increased membrane lipid peroxidation.
It can be inferred that in coastal China, C. fragile can improve light energy utilization (α) to increase the Fv/Fm of the thalli in winter when light intensity is reduced. Under the enhanced light intensity in summer, the damage caused by photoinhibition can be reduced or avoided by increasing the Ek and qP in C. fragile. The self-protection ability of C. cylindricum at low and high light intensities may be weaker than that of C. fragile. Under high light intensity, the photosynthetic activity of C. cylindricum decreases, and thus it disappears in mid-July along the southern coast of China. In winter, when light intensity is reduced, C. cylindricum can improve photosynthetic efficiency by increasing light energy utilization (α) and accumulating pigment content. In general, the adaptation to light differs between C. fragile and C. cylindricum. Compared to C. cylindricum, C. fragile is better adapted to different light intensities, especially at high light intensities, where C. fragile is significantly more adaptable than C. cylindricum. The results are to some extent consistent with the tidal distribution characteristics of these two species of Codium, with the C. fragile mostly distributed in the intertidal area with stronger light intensity and the C. cylindricum mostly distributed in the lower intertidal to subtidal area with weaker light intensity. At the same time, the results of this study can also explain why C. fragile can widely invade the world from the perspective of light intensity, while the C. cylindricum did not become a widespread invasive species. However, during the change of seasons, the temperature, salinity, and nitrogen source of the environment will also change, and temperature, light, salinity, and nitrogen source all have different degrees of influence on the growth of Codium (Hanisak, 1979). Therefore, to further understand the environmental adaptations of C. fragile under seasonal changes and the reasons why it can successfully invade worldwide, it is especially important to study the effects of other environmental factors such as temperature, salinity, and nutrient salts on it, which also points the way to our next work.
-
Figure 1. The Fv/Fm of two Codium species cultured at different light intensities. a. The Fv/Fm of C. fragile cultured at different light intensities, and b. the Fv/Fm of C. cylindricum cultured at different light intensities. Different letters (a, b, c, and d) represent significant differences among groups.
Figure 3. The rETRmax, photosynthetic rate in the light-limited region of RLC (α), and Ek of two Codium species cultured at different light intensities. The data were obtained from RLCs. a. The comparison of rETRmax of C. fragile cultured at different light intensities, b. the comparison of rETRmax of C. cylindricum cultured at different light intensities, c. the comparison of α of C. fragile cultured at different light intensities, d. the comparison of α of C. cylindricum cultured at different light intensities, e. the comparison of Ek of C. fragile cultured at different light intensities, and f. the comparison of Ek of C. cylindricum cultured at different light intensities. Different letters (a, b, and c) represent significant differences among groups.
Figure 8. The comparison of POD activity (a, letters a represent no significant differences among groups (p>0.05, ANOVA, followed by Tukey's multiple comparison test)) and T-AOC (b, different letters (a and b) represent significant differences among groups (p<0.05, ANOVA, followed by Tukey's multiple comparison test)) of two Codium species under different light intensities.
Table 1. Operational procedure for the determination of T-AOC
Measurement tube Control tube Reagent 1/mL 1 1 Sample to be tested/mL a* Reagent 2/mL 2 2 Reagent 3/mL 0.5 0.5 Mix thoroughly with a vortex mixer, 37℃ water bath for 30 min Reagent 4/mL 0.2 0.2 Sample to be tested/mL a* Reagent 5/mL 0.2 0.2 Note: The reference sampling volume for 10% tissue homogenate is 0.2 mL. -
Benson E E, Rutter J C, Cobb A H. 1983. Seasonal variation in frond morphology and chloroplast physiology of the intertidal alga Codium fragile (Suringar) Hariot. New Phytologist, 95(4): 569–580, doi: 10.1111/j.1469-8137.1983.tb03522.x Bird C J, Dadswell M J, Grund D W. 1993. First record of the potential nuisance alga Codium fragile ssp. tomentosoides (Chlorophyta, Caulerpales) in Atlantic Canada. Proceedings of the Nova Scotian Institute of Science, 40(1): 11–17 Bouman H A, Platt T, Doblin M, et al. 2018. Photosynthesis–irradiance parameters of marine phytoplankton: synthesis of a global data set. Earth System Science Data, 10(1): 251–266, doi: 10.5194/essd-10-251-2018 Carlton J T, Scanlon J A. 1985. Progression and dispersal of an introduced alga: Codium fragile ssp. tomentosoides (Chlorophyta) on the Atlantic coast of North America. Botanica Marina, 28(4): 155–166, doi: 10.1515/botm.1985.28.4.155 Chapman A S. 1998. From introduced species to invader: what determines variation in the success of Codium fragile ssp. tomentosoides (Chlorophyta) in the North Atlantic Ocean? Helgolä ander Meeresuntersuchungen, 52(3-4): 277–289 Churchill A C, Moeller H W. 1972. Seasonal patterns of reproduction in New York. Populations of Codium fragile (sur. ) Hariot subsp. tomentosoides (Van Goor) Silva. Journal of Phycology, 8(2): 147–152, doi: 10.1111/j.0022-3646.1972.00147.x Ciompi S, Gentili E, Guidi L, et al. 1996. The effect of nitrogen deficiency on leaf gas exchange and chlorophyll fluorescence parameters in sunflower. Plant Science, 118(2): 177–184, doi: 10.1016/0168-9452(96)04442-1 Ding Lanping, Wang Xulei, Huang Bingxin, et al. 2022. The environmental adaptability and reproductive properties of invasive green alga Codium fragile from the Nan'ao Island, South China Sea. Acta Oceanologica Sinica, 41(3): 70–75, doi: 10.1007/s13131-021-1928-6 Dromgoole F I. 1975. Occurrence of Codium fragile subspecies tomentosoides in New Zealand waters. New Zealand Journal of Marine and Freshwater Research, 9(3): 257–264, doi: 10.1080/00288330.1975.9515564 Drouin A, McKindsey C W, Johnson L E. 2012. Detecting the impacts of notorious invaders: experiments versus observations in the invasion of eelgrass meadows by the green seaweed Codium fragile. Oecologia, 168(2): 491–502, doi: 10.1007/s00442-011-2086-x Du Xiumin, Yin Wenxuan, Zhao Yanxiu, et al. 2001. The production and scavenging of reactive oxygen species in plants. Chinese Journal of Biotechnology (in Chinese), 17(2): 121–125 Gao Guang, Liu Yameng, Li Xinshu, et al. 2016. An ocean acidification acclimatised green tide alga is robust to changes of seawater carbon chemistry but vulnerable to light stress. PLoS One, 11(12): e0169040, doi: 10.1371/journal.pone.0169040 Gao Guang, Xu Zhiguang, Shi Qi, et al. 2018. Increased CO2 exacerbates the stress of ultraviolet radiation on photosystem II function in the diatom Thalassiosira weissflogii. Environmental and Experimental Botany, 156: 96–105, doi: 10.1016/j.envexpbot.2018.08.031 Goss R, Böhme K, Wilhelm C. 1998. The xanthophyll cycle of Mantoniella squamata converts violaxanthin into antheraxanthin but not to zeaxanthin: consequences for the mechanism of enhanced non-photochemical energy dissipation. Planta, 205(4): 613–621, doi: 10.1007/s004250050364 Guidi L, Degl’Innocenti E. 2012. Chlorophyll a fluorescence in abiotic stress. In: Venkateswarlu B, Shanker A K, Shanker C, et al. , eds. Crop Stress and Its Management: Perspectives and Strategies. Dordrecht: Springer: 359–398 Hanisak M D. 1979. Growth patterns of Codium fragile ssp. tomentosoides in response to temperature, irradiance, salinity, and nitrogen source. Marine Biology, 50(4): 319–332, doi: 10.1007/BF00387009 Hanisak M D, Harlin M M. 1978. Uptake of inorganic nitrogen by Codium fragile subsp. tomentosoides (Chlorophyta). Journal of Phycology, 14(4): 450–454, doi: 10.1111/j.1529-8817.1978.tb02467.x Hui Hongxia, Xu Xing, Li Shouming. 2004. Possible mechanism of inhibition on photosynthesis of Lycium barbarum under salt stress. Chinese Journal of Ecology (in Chinese), 23(1): 5–9 Israel A, Einav R, Silva P C, et al. 2010. First report of the seaweed Codium parvulum (Chlorophyta) in Mediterranean waters: recent blooms on the northern shores of Israel. Phycologia, 49(2): 107–112, doi: 10.2216/PH09-28.1 Lapointe B E, Barile P J, Littler M M, et al. 2005. Macroalgal blooms on southeast Florida coral reefs: I. Nutrient stoichiometry of the invasive green alga Codium isthmocladum in the wider Caribbean indicates nutrient enrichment. Harmful Algae, 4(6): 1092–1105, doi: 10.1016/j.hal.2005.06.004 Leandro A, Pereira L, Gonçalves A M M. 2020. Diverse applications of marine macroalgae. Marine Drugs, 18(1): 17 Ma Ting, Li Qiang, Wang Guoxiang, et al. 2006. Influence of suspended sands on rapid light curves of Ceratophyllum demersum in turbid solution. Journal of Wuhan Botanical Research (in Chinese), 24(6): 531–535 Magnusson M, Mata L, Wang Na, et al. 2015. Manipulating antioxidant content in macroalgae in intensive land-based cultivation systems for functional food applications. Algal Research, 8: 153–160, doi: 10.1016/j.algal.2015.02.007 Malinowski K C, Ramus J. 1973. Growth of the green alga Codium fragile in a Connecticut estuary. Journal of Phycology, 9(1): 102–110, doi: 10.1111/j.0022-3646.1973.00102.x Maoka T. 2020. Carotenoids as natural functional pigments. Journal of Natural Medicines, 74(1): 1–16, doi: 10.1007/s11418-019-01364-x Marques R, Cruz S, Calado R, et al. 2021. Effects of photoperiod and light spectra on growth and pigment composition of the green macroalga Codium tomentosum. Journal of Applied Phycology, 33(1): 471–480, doi: 10.1007/s10811-020-02289-9 Marques R, Moreira A, Cruz S, et al. 2022. Controlling light to optimize growth and added value of the green macroalga Codium tomentosum. Frontiers in Marine Science, 9: 906332, doi: 10.3389/fmars.2022.906332 Matsubara K, Matsuura Y, Bacic A, et al. 2001. Anticoagulant properties of a sulfated galactan preparation from a marine green alga, Codium cylindricum. International Journal of Biological Macromolecules, 28(5): 395–399, doi: 10.1016/S0141-8130(01)00137-4 Maxwell K, Johnson G N. 2000. Chlorophyll fluorescence—a practical guide. Journal of Experimental Botany, 51(345): 659–668, doi: 10.1093/jexbot/51.345.659 Neill P E, Alcalde O, Faugeron S, et al. 2006. Invasion of Codium fragile ssp. tomentosoides in northern Chile: a new threat for Gracilaria farming. Aquaculture, 259(1-4): 202–210, doi: 10.1016/j.aquaculture.2006.05.009 Parsons T R, Strickland J. 1963. Discussion of spectrophotometric determination of marine-plant pigments, with revised equations for ascertaining chlorophylls and carotenoids. Journal of Marine Research, 21(3): 155–163 Pérez-Cirera J L, Cremades J, Bárbara I. 1989. Systematic and synecologic comments about some new seaweed records for Galicia or for the Atlantic coasts of the Iberian Peninsula. Anales del Jardín Botánico de Madrid, 46(1): 35–45 Platt T, Gallegos C L, Harrison W G. 1980. Photoinhibition of photosynthesis in natural assemblages of marine phytoplankton. Journal of Marine Research, 38(4): 687–701 Porra R J. 2002. The chequered history of the development and use of simultaneous equations for the accurate determination of chlorophylls a and b. Photosynthesis Research, 73(1): 149–156 Ruban A V, Young A J, Pascal A A, et al. 1994. The effects of illumination on the xanthophyll composition of the photosystem II light-harvesting complexes of spinach thylakoid membranes. Plant Physiology, 104(1): 227–234, doi: 10.1104/pp.104.1.227 Schreiber U. 2004. Pulse-Amplitude-Modulation (PAM) fluorometry and saturation pulse method: an overview. In: Papageorgiou G C, Govindjee, eds. Chlorophyll A Fluorescence: A Signature of Photosynthesis. Dordrecht: Springer: 279–319 Silva P C. 1955. The dichotomous species of Codium in Britain. Journal of the Marine Biological Association of the United Kingdom, 34(3): 565–577, doi: 10.1017/S0025315400008821 Trowbridge C D. 1995. Establishment of the green alga Codium fragile ssp. tomentosoides on New Zealand rocky shores: current distribution and invertebrate grazers. Journal of Ecology, 83(6): 949–965, doi: 10.2307/2261177 Van Breusegem F, Vranová E, Dat J F, et al. 2001. The role of active oxygen species in plant signal transduction. Plant Science, 161(3): 405–414, doi: 10.1016/S0168-9452(01)00452-6 Velikova V, Yordanov I, Edreva A. 2000. Oxidative stress and some antioxidant systems in acid rain-treated bean plants: protective role of exogenous polyamines. Plant Science, 151(1): 59–66, doi: 10.1016/S0168-9452(99)00197-1 Vitousek P M, Mooney H A, Lubchenco J, et al. 1957. Human domination of Earth's ecosystems. Science, 277(5325): 494–499 Wang Hui-Min David, Chen Ching-Chun, Huynh P, et al. 2015. Exploring the potential of using algae in cosmetics. Bioresource Technology, 184: 355–362, doi: 10.1016/j.biortech.2014.12.001 Wei Liyuan, Zheng Yi. 2021. Effects of temperature and salinity on growth and nutrient absorption of Codium fragile Hariot. Current Biotechnology (in Chinese), 11(2): 190–195 Williams S L. 2007. Introduced species in seagrass ecosystems: status and concerns. Journal of Experimental Marine Biology and Ecology, 350(1-2): 89–110, doi: 10.1016/j.jembe.2007.05.032 Xu Daquan, Zhang Yuzhong, Zhang Rongxian. 1992. Photoinhibition of photosynthesis in plants. Plant Physiology Communications (in Chinese), 28(4): 237–243 Yan Shanglong, Pan Chuang, Yang Xianqing, et al. 2021. Degradation of Codium cylindricum polysaccharides by H2O2-Vc-ultrasonic and H2O2-Fe2+-ultrasonic treatment: structural characterization and antioxidant activity. International Journal of Biological Macromolecules, 182: 129–135, doi: 10.1016/j.ijbiomac.2021.03.193 Yang M H, Blunden G, Huang F L, et al. 1997. Growth of a dissociated, filamentous stage of Codium species in laboratory culture. Journal of Applied Phycology, 9(1): 1–3, doi: 10.1023/A:1007996207924 Yang Xiaoqing, Zhang Suiqi, Liang Zongsuo, et al. 2004. Effects of water stress on chlorophyll fluorescence parameters of different drought resistance winter wheat cultivars seedlings. Acta Botanica Boreali-Occidentalia Sinica (in Chinese), 24(5): 812–816 Ye Jincong, Ning Yue, Zeng Zhinan, et al. 2010. A study of both temperature and underwater illuminance effect on the growth of green alga, Codium fragile (Suringar) Hariot. Journal of Fujian Fisheries (in Chinese), (4): 25–28 Yin Shuaiwen, He Xumei, Lang Fengxiang. 2007. Study on bacteriostatic activity of Codium fragile. Journal of Jinggangshan University (Natural Sciences) (in Chinese), 28(8): 45–46 Zubia M, Robledo D, Freile-Pelegrin Y. 2007. Antioxidant activities in tropical marine macroalgae from the Yucatan Peninsula, Mexico. Journal of Applied Phycology, 19(5): 449–458, doi: 10.1007/s10811-006-9152-5 -
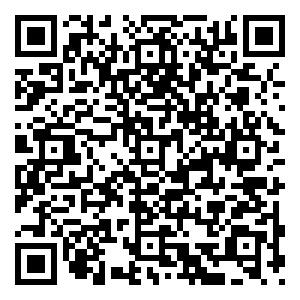
计量
- 文章访问数: 23
- HTML全文浏览量: 8
- 被引次数: 0