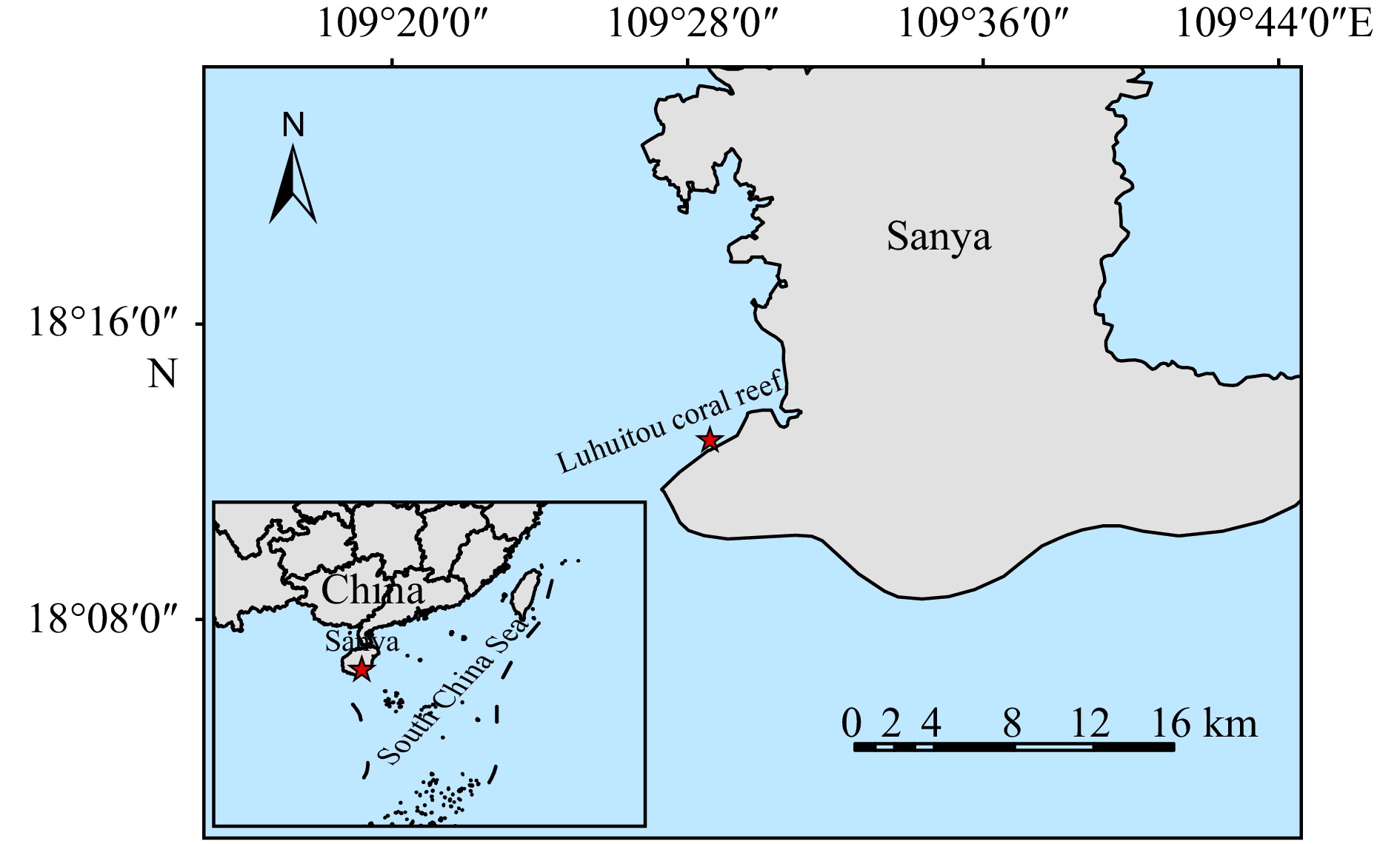
Citation: | Yi Huang, Cheng Feng, Bohai Gao, Lijuan Long, Fangfang Yang. Seasonal dynamics of Symbiodiniaceae communities associated with nine coral species in Luhuitou coral reef, South China Sea[J]. Acta Oceanologica Sinica. doi: 10.1007/s13131-024-2366-z |
Reef-building corals are crucial components of coral reef ecosystems, which can provide diverse and unique habitats that furnish food, shelter, and breeding grounds for diverse marine organisms (Hughes et al., 2017). The existence of reef-building corals relies on their mutualistic relationships with the symbiotic dinoflagellates within the family Symbiodiniaceae. Generally, these symbiotic Symbiodiniaceae can provide up to 95% of the coral host’s nutritional requirements through the translocation of photosynthetic products. Meanwhile, the metabolic byproducts of corals are transferred to symbiotic Symbiodiniaceae, serving as a source of inorganic nutrients for photosynthesis (Yellowlees et al., 2008). Several reports have highlighted the close association between Symbiodiniaceae species and coral responses to environmental stress. So far, there exist over 15 genera comprising hundreds of distinct genotypes within the Symbiodiniaceae family (LaJeunesse et al., 2018, 2022). Among these, Symbiodinium, Breviolum, Cladocopium, Durusdinium, Fugacium, and Gerakladium commonly exist as endosymbiont with reef-building corals (LaJeunesse, 2001). Corals containing Cladocopium bleached severely by expulsing algal symbionts, while corals containing Durusdinium were not affected after thermal stress event (Baker et al., 2004). Additionally, Symbiodiniaceae can exist freely in reef environments, which are important sources of symbionts for corals (Yamashita and Koike, 2013). It has been reported that approximately 85% of reef-building corals acquire their symbiotic Symbiodiniaceae from the environment (Baird et al., 2009). Therefore, understanding the diversity and compositions of symbiotic and free-living Symbiodiniaceae is crucial for comprehending the resilience and vulnerability of coral reef ecosystems.
The symbiotic relationship between Symbiodiniaceae and corals is particularly vulnerable to environmental changes (Hoegh-Guldberg et al., 2007). According to the adaptive bleaching hypothesis, corals can acquire new type of Symbiodiniaceae from the environment (switching) or change the relative abundance of Symbiodiniaceae within colonies (shuffling) to adapt, sustain and survive against environmental stressors (Baker, 2003). Symbiodiniaceae communities associated with Acropora palifera in Taiwan, China exhibited seasonal fluctuations (Chen et al., 2005). In a similar vein, Sawall et al. (2014) reported that the compositions of Symbiodiniaceae in coral Pocillopora verrucosa differed in summer and winter. However, several studies hold contrary perspectives, indicating that most corals experienced no alterations in their symbiotic algae under stressful environmental conditions (Goulet, 2006, 2007; Howells et al., 2020). Acropora humilis and P. damicornis maintained a stable Symbiodiniaceae community structure in different seasons (Jandang et al., 2022). Quek et al. (2023) found that Symbiodiniaceae communities in three coral species (i.e., Merulina ampliata, Pachyseris speciosa, and Porites lutea) in an equatorial reef remained stable across seasons. The different responses of symbiotic Symbiodiniaceae communities to environmental changes may be related to coral species. Hume et al. (2020) found that the Symbiodiniaceae communities in the Red Sea reef were primarily influenced by coral species, followed by water depth and seasonal factors. Similarly, in the Perhentian and Redang Islands Marine Park, the symbiotic Symbiodiniaceae communities were not affected by location or depth, but were significantly associated with the genus of coral hosts (Lee et al., 2022). Additionally, the diversity and temporal dynamics of free-living Symbiodiniaceae in coral reef environments remain largely unknown (Fujise et al., 2021; Gong et al., 2021). Fujise et al. (2021) discovered significant variations in the composition of free-living Symbiodiniaceae among diverse habitats and seasons within the southern Great Barrier Reef, Australia. Therefore, it is essential to study the variability of symbiotic Symbiodiniaceae in various species of corals and free-living Symbiodiniaceae to predict the potential responses of the reef community to environmental conditions.
The internal transcribed spacer 2 (ITS2) of nrDNA is one of the most widely used genetic marker to analyze Symbiodiniaceae diversity. High-throughput sequencing techniques are mainly used to characterize ITS2 sequence diversity, which can detect low abundance symbiotic Symbiodiniaceae genotypes in corals (Thomas et al., 2014). However, there may be more intra- and intergenomic sequence variation within a sample, posing challenges to species annotation. Recently, SymPortal analytical framework has been proposed for analyzing Symbiodiniaceae diversity due to superior taxonomic capability (Hume et al., 2019). It has demonstrated effectiveness in processing and analyzing high-throughput ITS2 sequencing data of Symbiodiniaceae, yielding more detailed and higher-resolution analytical results. Saad et al. (2022) analyzed the diversity of Symbiodiniaceae in corals in Hong Kong, China using the SymPortal framework and obtained 13 Symbiodiniaceae ITS2 type profiles, highlighting the mosaicism of symbiotic Symbiodiniaceae communities within coral assemblages, with different coral species exhibiting varying degrees of symbiont specificity. Fujise et al. (2021) used the SymPortal framework to analyze the diversity of coral symbiotic Symbiodiniaceae and free-living Symbiodiniaceae on coral reefs, providing insights into the ecological roles and potential contributions of free-living Symbiodiniaceae to coral reef ecosystems.
The Luhuitou coral reef, located in the northern part of the South China Sea, has a tropical monsoon climate and is affected by the alternation of seasonal climate. Previous studies have verified seasonal variations in Symbiodiniaceae associated with corals in the Luhuitou coral reef, but they mostly focused on the density and effective photochemical efficiency of Symbiodiniaceae, rather than Symbiodiniaceae compositions (Xu et al., 2017; Tong et al., 2017; Gong et al., 2018). Moreover, ITS2 amplicon sequencing analysis has been applied in these studies. To date, high-throughput sequencing technologies and the SymPortal analytical framework have yet to be utilized in exploring the seasonal changes in the diversity and composition of both symbiotic and free-living Symbiodiniaceae in the Luhuitou coral reef. In this study, we applied SymPortal analytical framework to study the dynamics of Symbiodiniaceae diversity and composition in nine corals and seawater in response to seasonal variations in the Luhuitou coral reef. Furthermore, the impacts of seasonal environmental parameters on Symbiodiniaceae community structure and diversity were explored. This study provides an important basis for better understanding Symbiodiniaceae dynamics in the South China Sea, and contribute to predict the future of coral reef and protect them.
Corals (Pocillopora damicornis, Porites lutea, Hydnophora exesa, Goniastrea retiformis, Montipora truncate, M. aequituberculata, Acropora latistella, A. digitifera, A. divaricata) and seawater samples were collected from the Luhuitou coral reef, Hainan Province, China (18°12′43.556″N, 109°28′26.013″E) on October 22, 2021 (autumn), January 12, 2022 (winter), May 10, 2022 (spring), and July 27, 2022 (summer), respectively (Fig. 1). The corals were identified based on morphology identification (Fig. S1). Each coral species had at least three replicates. Coral samples were collected at a depth of about 2-4 m, and washed with 0.22 μm filtered seawater to remove loosely attached algae. The coral tissue was then rinsed out with Waterpik (GT3, America) equipped with sterilized seawater and filtered using 0.22 μm membranes (PALL,
Environmental variables of sampling sites were measured in four seasons, namely sea surface temperature (SST), salinity, pH, dissolved oxygen (DO), and nutrient concentration (nitrite (NO2-N), nitrate (NO3-N), ammonium (NH4-N), phosphate (PO4-P)). Each parameter was measured at least three times (n≥3). The sea surface temperature, salinity, pH and DO were measured by YSI Professional Plus (YSI Incorporated, USA) multi-parameter water quality detector. The YSI ProPlus is a portable multi-parameter water quality analyzer suitable for quickly and accurately measuring water quality parameters in freshwater, seawater, and wastewater. Nutrient concentrations of filtered seawater were determined by CleverChem380Plus automatic chemical analyzer (DeChem-Tech, Germany).
Total genomic DNA was extracted from coral and seawater samples using the FastDNA® Spin Kit for Soil (MP Biomedicals, U.S.) following the manufacturer’s instructions. The quality of extracted genomic DNA was detected by 1% agarose gel electrophoresis, and the DNA concentration and purity were determined by NanoDrop® ND-2000 spectrophotometer (Thermo Scientific Inc., USA). Using the extracted DNA as template, the ITS2 gene of Symbiodiniaceae was amplified with primer pairs F (5′-GAATTGCAGAACTCCGTG-3′) and R (5′-GGGATCCATATGCTTAAGTTCAGCGGGT-3′) by an ABI GeneAmp® 9700 PCR thermocycler (ABI, CA, USA). PCR amplification cycling conditions were as follows: denaturation was carried out at 95°C for 180 s, followed by denaturation at 95°C for 30 s, annealing at 55℃ for 30 s and extension at 72℃ for 45 s, and single extension at 72℃ for 10 min, and end at 4℃. All samples were amplified in triplicate. The PCR product was extracted from 2% agarose gel, purified using the AxyPrep DNA Gel Extraction Kit (Axygen Biosciences, Union City, CA, USA), and quantified using QuantusTM Fluorometer (Promega, USA). The purified amplicons were sent to Majorbio Bio-Pharm Technology Co. Ltd. (Shanghai, China) and paired-end sequenced on an Illumina MiSeq PE300 platform/NovaSeq PE250 platform (Illumina, San Diego, USA). The raw data were submitted to the NCBI Sequence Read Archive (accession number: PRJNA1012458).
ITS2 high-throughput sequencing data were analyzed using the local SymPortal framework-0.3.21 (Hume et al., 2019). Briefly, demultiplexed forward and reverse Fastq files were loaded into SymPortal for sequence quality control using MOTHUR 1.39.5 (Schloss et al., 2009), BLAST + suite (Camacho et al., 2009), and minimum entropy decomposition (MED) (Eren et al., 2015). Then SymPortal aligned the sample sequences with the SymPortal analysis database to identify defining intragenomic variants (DIVs), which were used to identify Symbiodiniaceae ITS2 type profile. The post-MED ITS2 sequences represent the assumed haplotypes and the ITS2 type profile represent taxa of Symbiodiniaceae.
All data analyses were performed in R version 4.1.1. The alpha and beta diversity of Symbiodiniaceae were calculated based on post-MED ITS2 sequences absolute abundance count table. The Shannon diversity index was calculated using the diversity analysis package vegan and the statistical analysis was performed using the stats and dunn.test packages in R. Differential analysis of Symbiodiniaceae ITS2 sequence type community composition was performed using non-metric multidimensional scaling (NMDS), Permutational Multivariate Analysis of Variance (PERMANOVA) and analysis of similarities (ANOSIM). The relationship between Symbiodiniaceae diversity and environmental variables was analyzed using Canonical Correspondence Analysis. Hierarchical clustering analysis was performed using Bray-Curtis distance and average linkage method, and the results were visualized using the pheatmap package. The sample correlation was calculated using the Hmisc package, and a network structure was constructed using the igraph package. Visualize the network structure using the Gephi 0.10.1 software.
A total of nine species of corals were collected from the Luhuitou coral reef in four seasons. All coral and seawater samples, except for the A. divaricata samples collected in autumn, were successfully sequenced. A total of 9 175 499 raw sequence reads were obtained from high-throughput sequencing of 154 samples. Following sequence quality control and minimum entropy decomposition in SymPortal framework-0.3.21, a total of 6 332 530 ITS2 sequence reads of Symbiodiniaceae were obtained, including 521 Symbiodiniaceae ITS2 sequence types (Fig. 2c). After identifying defining intragenomic variants (DIVs), 37 Symbiodiniaceae ITS2 type profiles (Fig. 2b) and 27 Symbiodiniaceae majority ITS2 sequences (the most abundant ITS2 sequence in a given ITS2 type profile) were obtained.
Three genera of Symbiodiniaceae were detected from 154 samples, among which Cladocopium was the most abundant (80.94%), followed by Durusdinium (19.05%), and Breviolum (0.01%) (Fig. 2a). Pocillopora damicornis was mainly dominated by Durusdinium (99.79%), while Porites lutea, Goniastrea retiformis, Montipora truncate, M. aequituberculata and A. divaricate were strongly associated with Cladocopium (100%). For Hydnophora exesa, Acropora latistella, A. digitifera, and seawater samples, Cladocopium and Durusdinium were detected. Additionally, there was limited exchange of Symbiodiniaceae between the seawater column and corals. Although 100 ITS2 sequence types and 11 ITS2 type profiles were identified in both corals and seawater (Fig. S2), only 4 ITS2 sequence types (C3u, C15, C1, and D1) and 1 ITS2 type profile (C21a-C21-C21k-C3-2033) had abundances exceeding 5%. Moreover, the main Symbiodiniaceae ITS2 sequence type was C15 in four seasons, while ITS2 type profile differed: C3u/C3 was predominant in autumn (16.24%), C1-C1c-C72k in winter (12.82%), C3/C50c/C50a-C3b-
The Shannon diversity of Symbiodiniaceae ITS2 sequence types from 154 samples ranged from 0.75 to 2.90, with no significant difference in the four seasons (p>0.05) (Fig. 3a), while significant differences were observed among various coral species and seawater (p<0.05, Fig. 3b). The highest average Shannon diversity was observed in A. latistella with a value of 2.59, followed by seawater with 2.32. On the other hand, the lowest average Shannon diversity was recorded in G. retiformis with a value of 0.88. Additionally, the seasonal variations in the Shannon diversity were different for various coral species. Shannon diversity of H. exesa, A. latistella, and A. divaricata had no significant seasonal variation (p>0.05), while other corals and seawater had significant seasonal variation (p<0.05) (Fig. 3b).
Overall, there was no significant difference in the Symbiodiniaceae community compositions based on ITS2 sequence types among the four seasons (Fig. 4a, Table 1). However, significant seasonal differences were observed in the Symbiodiniaceae community composition for each coral species and seawater (Table 2, Table S1. The pairwise comparison revealed that P. damicornis and P. lutea exhibited significant differences between any two seasons (Table S1). Furthermore, Symbiodiniaceae communities were influenced by coral host, followed by seasonal factors (Fig. 4b, Table 1). Additionally, significant differences were found in the Symbiodiniaceae communities among coral species, with the most pronounced difference between G. retiformis and A. divaricate (Table 1). Clear distinctions were also observed between Symbiodiniaceae associated with corals and those present in the water column (Table 1).
Factors | Variation (R2) | F. Model | p | |
Seasons | 0.023 | 1.04 | ||
Coral species | 0.80 | 66.19 | * | |
Corals vs seawater | 0.042 | 6.60 | * | |
P. damicornis vs P. lutea | 0.95 | 534.33 | * | |
P. damicornis vs H. exesa | 0.57 | 37.10 | * | |
P. damicornis vs G. retiformis | 0.96 | 752.17 | * | |
P. damicornis vs M. truncata | 0.95 | 541.33 | * | |
P. damicornis vs M. aequituberculata | 0.84 | 150.07 | * | |
P. damicornis vs A. latistella | 0.68 | 62.06 | * | |
P. damicornis vs A. digitifera | 0.54 | 33.81 | * | |
P. damicornis vs A. divaricata | 0.96 | 602.92 | * | |
P. damicornis vs seawater | 0.43 | 21.78 | * | |
P. lutea vs H. exesa | 0.68 | 60.80 | * | |
P. lutea vs G. retiformis | 0.98 | * | ||
P. lutea vs M. truncata | 0.97 | 850.15 | * | |
P. lutea vs M. aequituberculata | 0.64 | 52.74 | * | |
P. lutea vs A. latistella | 0.73 | 82.34 | * | |
P. lutea vs A. digitifera | 0.56 | 37.56 | * | |
P. lutea vs A. divaricata | 0.98 | * | ||
P. lutea vs seawater | 0.62 | 49.09 | * | |
H. exesa vs G. retiformis | 0.60 | 43.25 | * | |
H. exesa vs M. truncata | 0.66 | 57.20 | * | |
H. exesa vs M. aequituberculata | 0.59 | 41.80 | * | |
H. exesa vs A. latistella | 0.41 | 20.49 | * | |
H. exesa vs A. digitifera | 0.29 | 11.94 | * | |
H. exesa vs A. divaricata | 0.65 | 47.05 | * | |
H. exesa vs seawater | 0.25 | 9.48 | * | |
G. retiformis vs M. truncata | 0.98 | * | ||
G. retiformis vs M. aequituberculata | 0.87 | 199.17 | * | |
G. retiformis vs A. latistella | 0.67 | 62.26 | * | |
G.retiformis vs A. digitifera | 0.47 | 26.81 | * | |
G. retiformis vs A. divaricata | 0.99 | * | ||
G. retiformis vs seawater | 0.62 | 48.99 | * | |
M. truncata vs M. aequituberculata | 0.85 | 172.06 | * | |
M. truncata vs A. latistella | 0.72 | 78.23 | * | |
M. truncata vs A. digitifera | 0.55 | 36.40 | * | |
M. truncata vs A. divaricata | 0.98 | * | ||
M. truncata vs seawater | 0.67 | 60.13 | * | |
M. aequituberculata vs A. latistella | 0.64 | 53.90 | * | |
M. aequituberculata vs A. digitifera | 0.48 | 28.01 | * | |
M. aequituberculata vs A. divaricata | 0.85 | 151.02 | * | |
M. aequituberculata vs seawater | 0.52 | 32.97 | * | |
A. latistella vs A. digitifera | 0.076 | 2.46 | * | |
A. latistella vs A. divaricata | 0.71 | 63.22 | * | |
A. latistella vs seawater | 0.42 | 21.79 | * | |
A. digitifera vs A. divaricata | 0.47 | 23.48 | * | |
A. digitifera vs seawater | 0.30 | 13.04 | * | |
A. divaricata vs seawater | 0.57 | 34.65 | * | |
Note: The asterisk (*) indicates that the p value is less than 0.05. |
Factors | ANOSIM statistic R | Significance | |
P. damicornis | 0.001 | * | |
P. lutea | 0.001 | * | |
H. exesa | 0.001 | * | |
G. retiformis | 0.001 | * | |
M. truncata | 0.001 | * | |
M. aequituberculata | 0.004 | * | |
A. latistella | 0.001 | * | |
A. digitifera | 0.001 | * | |
A. divaricata | 0.034 | * | |
Seawater | 0.001 | * | |
Note: The asterisk (*) indicates that the significance value is less than 0.05. |
Canonical correspondence analysis was used to analyze the relationship between Symbiodiniaceae composition and environmental variables (sea surface temperature, pH, dissolved oxygen, nutrient concentrations). The environmental data for each season were shown in Table 3. The results showed that the compositions of Symbiodiniaceae associated with corals, excluding A. divaricata and M. aequituberculata, were significantly influenced by environmental factors (Fig. 5). Different coral species exhibit varying responses to environmental changes. Specifically, ammonium, nitrite, nitrate, phosphate, and SST exhibited significant effects on the compositions of Symbiodiniaceae. Nitrate exerted the most substantial influence on Symbiodiniaceae composition in P. damicornis, P. lutea, H. exesa, G. retiformis, and A. latistella. Ammonium and SST had notable impacts on Symbiodiniaceae compositions in M. truncata and A. digitifera. Additionally, the composition of Symbiodiniaceae in seawater showed significant associations with four environmental factors: ammonium, nitrate, phosphate, and SST.
Autumn | Winter | Spring | Summer | |
pH | 8.29 ± 0.16 | 8.12 ± 0.27 | 8.21 ± 0.24 | 8.07 ± 0.17 |
SST/℃ | 28.28 ± 1.13 | 24.03 ± 1.94 | 29.88 ± 1.36 | 30.63 ± 0.90 |
DO/mg·L−1 | 8.92 ± 2.92 | 7.48 ± 2.05 | 8.88 ± 2.22 | 7.75 ± 2.26 |
Nitrite/μg·L−1 | 3.72 ± 0.86 | 3.08 ± 0.54 | 1.79 ± 1.15 | 1.23 ± 0.22 |
Nitrate/μg·L−1 | 58.5 ± 5.07 | 26.5 ± 0.81 | 25.6 ± 5.17 | 23.3 ± 2.51 |
Ammonium/μg·L−1 | 30.3 ± 2.00 | 9.80 ± 0.77 | 35.5 ± 4.20 | 17.3 ± 1.35 |
Phosphate/μg·L−1 | 1.83 ± 1.44 | 0.286 ± 0.10 | 2.96 ± 2.42 | 4.48 ± 0.36 |
Based on the Bray-Curtis dissimilarity and average linkage method, 154 samples of Symbiodiniaceae ITS2 sequence types (excluding sequence types with relative abundance less than or equal to 0.05 in all samples) were hierarchical clustered into seven clusters (Fig. 6). Cluster 1 consisted of P. lutea and M. aequituberculata, in which C15 was the main Symbiodiniaceae ITS2 sequence types. Cluster 2 consisted of M. truncata, dominated by the C17f type. Cluster 3 was dominated by the C3u type, consisting of mainly G. retiformis. Cluster 5 mainly consisted of A. divaricata, dominated by the C1 type. Cluster 6 mainly consisted of P. damicornis and seawater, in which D1 type was dominant. Cluster 7 mainly consisted of A. latistella, A. digitifera, H. exesa, and seawater (winter), dominated by C3, C50a, C50c, C21a, and C21 types.
Based on hierarchical cluster analysis, the network interactions of seven clusters Symbiodiniaceae ITS2 sequence types (excluding sequence types with relative abundance less than or equal to 0.05 in all samples) were studied (Fig. 7). In network interaction analysis, Symbiodiniaceae ITS2 sequence types with correlation coefficients below 0.7 and significant p-values less than 0.05 were excluded. Overall, 65 nodes and 97 interactions were identified, of which 79 were co-occurrence (81.4% of the total) and 18 were co-exclusive (18.6% of the total). Cluster 1 network identified 9 nodes and 22 interactions. The major ITS2 sequence type was C15 (with an average abundance of 73.8%), which showed a high degree of co-exclusive relationships with other types. The major ITS2 sequence type of Cluster 2 network contained 3 nodes and 3 interactions was C17f (with an average abundance of 83.2%). The main interaction of C17f was co-exclusive. Cluster 3 network identified 4 nodes and 2 interactions. The major ITS2 sequence types of Cluster 3 were C3u and C3 (with average abundances of 80.6% and 17.1%, respectively), and their interaction was co-exclusive. In Cluster 4 and Cluster 5, no significant interactions among all ITS2 sequence types were observed. 28 nodes and 43 interactions were yield in Cluster 6 network, of which 84% were co-occurrence. The major ITS2 sequence types of Cluster 6 were D1 and D4 (with average abundances of 45.9% and 11.4%, respectively). In Cluster 6, there was no significant interaction detected for D1, while the interaction of D4 was co-exclusive. Cluster 7 network that mainly consist of C3, C21a and C50c identified 21 nodes and 27 interactions, of which 93% were co-occurrence and 7% were co-exclusive. C21a had a co-occurrence relationship with C21 and C21k, while C50c and C21k were mutually exclusive.
The Symbiodiniaceae family, comprising over 15 genera with hundreds of distinct genotypes, is crucial for coral reef ecosystems. Generally, scleractinian corals form symbioses with a main Symbiodiniaceae genus, such as Breviolum, Cladocopium or Durusdinium, although several corals may harbor various Symbiodiniaceae types simultaneously (Varasteh et al., 2021; de Souza et al., 2023). In our study, Pocillopora damicornis was dominated by the genus Durusdinium, while Cladocopium was main genus in other coral species. Furthermore, the dominant Symbiodiniaceae types were exclusive in the community, while rare Symbiodiniaceae types were mostly co-occurring based on the network analysis. Dominant Symbiodiniaceae types may enhance the stability of Symbiodiniaceae communities by suppressing rare Symbiodiniaceae types. Similarly, it has been reported that a key species, Durusdinium trenchii, contributed most co-exclusion relationships in Symbiodiniaceae interaction network of tropical South China Sea (Chen et al., 2020). These findings may also explain why individual corals can harbor multiple Symbiodiniaceae taxa, but usually only one Symbiodiniaceae taxa dominates.
Several studies have demonstrated that the compositions of Symbiodiniaceae are influenced by coral host species and environmental factors, including sea surface temperature, nitrogen, phosphorus (Gong et al., 2018; Chen et al., 2019, 2021). However, yet little have assessed how Symbiodiniaceae communities hosted by corals vary across seasons in the Luhuitou reef. In this study, the diversity and composition of Symbiodiniaceae communities were primarily influenced by biological factors, species of coral host, with seasonal factors exerting a secondary influence. Similar results were reported that that Symbiodiniaceae community in the Red Sea reefs was mainly driven by coral species, followed by depth and season (Hume et al., 2020). In the Perhentian and Redang Islands Marine Parks, Symbiodiniaceae communities were not influenced by location or depth, but they were significantly affected by the genera of coral hosts (Lee et al., 2022). However, Chen et al. (2019) analyzed the Symbiodiniaceae compositions from eight coral habitats in the South China Sea, and found that coral species had little effect on the dominant Symbiodiniaceae types, with the composition primarily driven by environmental factors. The different results may be due to coral species or environment parameters at sampling sites.
It is worth noting that, in this study, the compositions of Symbiodiniaceae communities associated with each species of corals, excluding A. divaricata and M. aequituberculata, were significantly influenced by environmental factors (i.e., sea surface temperature and nutrient concentrations). This aligns with findings from several studies that have reported the influence of temperature (SST) and nutrient on the coral-Symbiodiniaceae symbiotic relationships (Gong et al., 2018; Chen et al., 2019). The significant correlation between environmental factors and Symbiodiniaceae compositions may be a result of different types of Symbiodiniaceae adapting to the environment. Previous studies have highlighted variations in temperature tolerance among different Symbiodiniaceae types, with Durusdinium, for example, being regarded as a heat-tolerant Symbiodiniaceae (Abrego et al., 2008; Swain et al., 2017; Gong et al., 2019). We observed a significant decrease in the relative abundance of Durusdinium in the seawater column during winter. This finding further supports the notion that different types of Symbiodiniaceae exhibit distinct growth preferences and occupy different ecological niches. A study revealed that when the sea water temperature rose, coral host cells experienced minimal or no deaths, whereas the levels of apoptosis and necrosis in symbiotic Symbiodiniaceae cells increased significantly (Strychar and Sammarco, 2009). This indicated that the impact of high temperature on coral was primarily due to the sensitivity of the symbiotic Symbiodiniaceae to temperature changes. Under heat stress conditions, different types of Symbiodiniaceae produced varying levels of reactive oxygen species (ROS), and their antioxidant component composition and activity also exhibited significant differences (Krueger et al., 2014). Consequently, the different tolerance levels of various Symbiodiniaceae types to temperature may lead to changes in the composition of coral symbiotic Symbiodiniaceae. Additionally, study has shown that the enrichment of seawater nutrients affects the stability of the symbiotic relationship between coral and Symbiodiniaceae, increasing the sensitivity of coral bleaching (Donovan et al., 2020). Various Symbiodiniaceae types have different abilities to absorb nitrogen nutrients. Under non-stress conditions, Cladocopium absorbed more nitrogen than Durusdinium, which might explain Cladocopium maintaining their dominance globally by allowing them to outcompete other Symbiodiniaceae for limited nutrients over time (Baker et al., 2013). Corals may adapt to changes in nutrient environments by adjusting the composition of their symbiotic Symbiodiniaceae communities. Therefore, management actions should be taken to reduce the coastal eutrophication and global climate change to avoid coral bleaching.
Corals can improve their adaptability to environment stresses by modulate their Symbiodiniaceae background types. In our study, the dominant ITS2 sequence types of Symbiodiniaceae remained relatively stable for each species throughout our study, even though seasonal variations contributed to the diversity and compositions of Symbiodiniaceae. It has been speculated that corals may modulate their Symbiodiniaceae background types to copy with different seasonal conditions. Rare background Symbiodiniaceae members may made significant contributions to the stability of the host- Symbiodiniaceae community and may play important roles in the adaptive capacity of the coral to environmental changes (Ziegler et al., 2018). Similarly, coral A. valida didn’t change the dominant Symbiodiniaceae types under ocean acidification conditions, while the relative abundance of background types of Symbiodiniaceae increased, implying that corals may regulate the rare biosphere of Symbiodiniaceae to enhance the stability of the symbiotic relationship between corals and Symbiodiniaceae, allowing them to adapt to acidic environments (Ge et al., 2021).
Free-living Symbiodiniaceae are regarded as a significant source pool for coral symbiotic Symbiodiniaceae, since approximately 85% of corals acquire their symbiotic Symbiodiniaceae from the environment (Coffroth et al., 2006). Several corals can increase survival by developing symbiotic relationships with more tolerant free-living Symbiodiniaceae from the surrounding water (Fabina et al., 2013). However, Huang et al. (2013) discovered Symbiodiniaceae types hosted by corals were completely different from those in the adjacent seawater in the Luhuitou coral reef, and speculated that corals did not recruit Symbiodiniaceae from the surrounding environment. Similarly, we found that there were significant differences in the Symbiodiniaceae ITS2 sequence types between coral species and surrounding seawater in the Luhuitou coral reef. Free-living Symbiodiniaceae can be found not only in the seawater but also on the surfaces of macroalgae and sediments, serving as potential sources of Symbiodiniaceae for corals (Yamashita and Koike, 2013). Therefore, further research is needed to explore the relationship between free-living Symbiodiniaceae in the various environment and symbiotic Symbiodiniaceae in corals.
Our study provides valuable insights into the species and seasonal variation in Symbiodiniaceae communities in the Luhuitou coral reef. The research found that Symbiodintaceae community was primarily influenced with its host species, followed by seasonal variations. We observed the ability of corals to adapt and modify their symbiotic associations in response to seasonal fluctuations. Among the studied coral species, P. damicornis and P. lutea exhibited the greatest seasonal variation in Symbiodiniaceae composition, primarily driven by changes in the rare Symbiodiniaceae composition. Furthermore, we observed significant differences between the composition of symbiotic Symbiodiniaceae in corals and free-living Symbiodiniaceae in the seawater. However, they were not entirely independent, although the relative abundances of shared Symbiodiniaceae types were relatively low. This study also found that SST and nutrients (nitrite, nitrate, ammonium, phosphate) were vital environmental factors that influence the Symbiodiniaceae compositions. The network analysis revealed that the dominant Symbiodiniaceae types in the community were likely maintained through competitive mechanisms to ensure community stability. These findings contribute to conservation and protection of coral reef ecosystems.
Factors | Variation (R2) | F. Model | p | |
Seasons | 0.023 | 1.04 | ||
Coral species | 0.80 | 66.19 | * | |
Corals vs seawater | 0.042 | 6.60 | * | |
P. damicornis vs P. lutea | 0.95 | 534.33 | * | |
P. damicornis vs H. exesa | 0.57 | 37.10 | * | |
P. damicornis vs G. retiformis | 0.96 | 752.17 | * | |
P. damicornis vs M. truncata | 0.95 | 541.33 | * | |
P. damicornis vs M. aequituberculata | 0.84 | 150.07 | * | |
P. damicornis vs A. latistella | 0.68 | 62.06 | * | |
P. damicornis vs A. digitifera | 0.54 | 33.81 | * | |
P. damicornis vs A. divaricata | 0.96 | 602.92 | * | |
P. damicornis vs seawater | 0.43 | 21.78 | * | |
P. lutea vs H. exesa | 0.68 | 60.80 | * | |
P. lutea vs G. retiformis | 0.98 | * | ||
P. lutea vs M. truncata | 0.97 | 850.15 | * | |
P. lutea vs M. aequituberculata | 0.64 | 52.74 | * | |
P. lutea vs A. latistella | 0.73 | 82.34 | * | |
P. lutea vs A. digitifera | 0.56 | 37.56 | * | |
P. lutea vs A. divaricata | 0.98 | * | ||
P. lutea vs seawater | 0.62 | 49.09 | * | |
H. exesa vs G. retiformis | 0.60 | 43.25 | * | |
H. exesa vs M. truncata | 0.66 | 57.20 | * | |
H. exesa vs M. aequituberculata | 0.59 | 41.80 | * | |
H. exesa vs A. latistella | 0.41 | 20.49 | * | |
H. exesa vs A. digitifera | 0.29 | 11.94 | * | |
H. exesa vs A. divaricata | 0.65 | 47.05 | * | |
H. exesa vs seawater | 0.25 | 9.48 | * | |
G. retiformis vs M. truncata | 0.98 | * | ||
G. retiformis vs M. aequituberculata | 0.87 | 199.17 | * | |
G. retiformis vs A. latistella | 0.67 | 62.26 | * | |
G.retiformis vs A. digitifera | 0.47 | 26.81 | * | |
G. retiformis vs A. divaricata | 0.99 | * | ||
G. retiformis vs seawater | 0.62 | 48.99 | * | |
M. truncata vs M. aequituberculata | 0.85 | 172.06 | * | |
M. truncata vs A. latistella | 0.72 | 78.23 | * | |
M. truncata vs A. digitifera | 0.55 | 36.40 | * | |
M. truncata vs A. divaricata | 0.98 | * | ||
M. truncata vs seawater | 0.67 | 60.13 | * | |
M. aequituberculata vs A. latistella | 0.64 | 53.90 | * | |
M. aequituberculata vs A. digitifera | 0.48 | 28.01 | * | |
M. aequituberculata vs A. divaricata | 0.85 | 151.02 | * | |
M. aequituberculata vs seawater | 0.52 | 32.97 | * | |
A. latistella vs A. digitifera | 0.076 | 2.46 | * | |
A. latistella vs A. divaricata | 0.71 | 63.22 | * | |
A. latistella vs seawater | 0.42 | 21.79 | * | |
A. digitifera vs A. divaricata | 0.47 | 23.48 | * | |
A. digitifera vs seawater | 0.30 | 13.04 | * | |
A. divaricata vs seawater | 0.57 | 34.65 | * | |
Note: The asterisk (*) indicates that the p value is less than 0.05. |
Factors | ANOSIM statistic R | Significance | |
P. damicornis | 0.001 | * | |
P. lutea | 0.001 | * | |
H. exesa | 0.001 | * | |
G. retiformis | 0.001 | * | |
M. truncata | 0.001 | * | |
M. aequituberculata | 0.004 | * | |
A. latistella | 0.001 | * | |
A. digitifera | 0.001 | * | |
A. divaricata | 0.034 | * | |
Seawater | 0.001 | * | |
Note: The asterisk (*) indicates that the significance value is less than 0.05. |
Autumn | Winter | Spring | Summer | |
pH | 8.29 ± 0.16 | 8.12 ± 0.27 | 8.21 ± 0.24 | 8.07 ± 0.17 |
SST/℃ | 28.28 ± 1.13 | 24.03 ± 1.94 | 29.88 ± 1.36 | 30.63 ± 0.90 |
DO/mg·L−1 | 8.92 ± 2.92 | 7.48 ± 2.05 | 8.88 ± 2.22 | 7.75 ± 2.26 |
Nitrite/μg·L−1 | 3.72 ± 0.86 | 3.08 ± 0.54 | 1.79 ± 1.15 | 1.23 ± 0.22 |
Nitrate/μg·L−1 | 58.5 ± 5.07 | 26.5 ± 0.81 | 25.6 ± 5.17 | 23.3 ± 2.51 |
Ammonium/μg·L−1 | 30.3 ± 2.00 | 9.80 ± 0.77 | 35.5 ± 4.20 | 17.3 ± 1.35 |
Phosphate/μg·L−1 | 1.83 ± 1.44 | 0.286 ± 0.10 | 2.96 ± 2.42 | 4.48 ± 0.36 |
Factors | Variation (R2) | F. Model | p | |
Seasons | 0.023 | 1.04 | ||
Coral species | 0.80 | 66.19 | * | |
Corals vs seawater | 0.042 | 6.60 | * | |
P. damicornis vs P. lutea | 0.95 | 534.33 | * | |
P. damicornis vs H. exesa | 0.57 | 37.10 | * | |
P. damicornis vs G. retiformis | 0.96 | 752.17 | * | |
P. damicornis vs M. truncata | 0.95 | 541.33 | * | |
P. damicornis vs M. aequituberculata | 0.84 | 150.07 | * | |
P. damicornis vs A. latistella | 0.68 | 62.06 | * | |
P. damicornis vs A. digitifera | 0.54 | 33.81 | * | |
P. damicornis vs A. divaricata | 0.96 | 602.92 | * | |
P. damicornis vs seawater | 0.43 | 21.78 | * | |
P. lutea vs H. exesa | 0.68 | 60.80 | * | |
P. lutea vs G. retiformis | 0.98 | * | ||
P. lutea vs M. truncata | 0.97 | 850.15 | * | |
P. lutea vs M. aequituberculata | 0.64 | 52.74 | * | |
P. lutea vs A. latistella | 0.73 | 82.34 | * | |
P. lutea vs A. digitifera | 0.56 | 37.56 | * | |
P. lutea vs A. divaricata | 0.98 | * | ||
P. lutea vs seawater | 0.62 | 49.09 | * | |
H. exesa vs G. retiformis | 0.60 | 43.25 | * | |
H. exesa vs M. truncata | 0.66 | 57.20 | * | |
H. exesa vs M. aequituberculata | 0.59 | 41.80 | * | |
H. exesa vs A. latistella | 0.41 | 20.49 | * | |
H. exesa vs A. digitifera | 0.29 | 11.94 | * | |
H. exesa vs A. divaricata | 0.65 | 47.05 | * | |
H. exesa vs seawater | 0.25 | 9.48 | * | |
G. retiformis vs M. truncata | 0.98 | * | ||
G. retiformis vs M. aequituberculata | 0.87 | 199.17 | * | |
G. retiformis vs A. latistella | 0.67 | 62.26 | * | |
G.retiformis vs A. digitifera | 0.47 | 26.81 | * | |
G. retiformis vs A. divaricata | 0.99 | * | ||
G. retiformis vs seawater | 0.62 | 48.99 | * | |
M. truncata vs M. aequituberculata | 0.85 | 172.06 | * | |
M. truncata vs A. latistella | 0.72 | 78.23 | * | |
M. truncata vs A. digitifera | 0.55 | 36.40 | * | |
M. truncata vs A. divaricata | 0.98 | * | ||
M. truncata vs seawater | 0.67 | 60.13 | * | |
M. aequituberculata vs A. latistella | 0.64 | 53.90 | * | |
M. aequituberculata vs A. digitifera | 0.48 | 28.01 | * | |
M. aequituberculata vs A. divaricata | 0.85 | 151.02 | * | |
M. aequituberculata vs seawater | 0.52 | 32.97 | * | |
A. latistella vs A. digitifera | 0.076 | 2.46 | * | |
A. latistella vs A. divaricata | 0.71 | 63.22 | * | |
A. latistella vs seawater | 0.42 | 21.79 | * | |
A. digitifera vs A. divaricata | 0.47 | 23.48 | * | |
A. digitifera vs seawater | 0.30 | 13.04 | * | |
A. divaricata vs seawater | 0.57 | 34.65 | * | |
Note: The asterisk (*) indicates that the p value is less than 0.05. |
Factors | ANOSIM statistic R | Significance | |
P. damicornis | 0.001 | * | |
P. lutea | 0.001 | * | |
H. exesa | 0.001 | * | |
G. retiformis | 0.001 | * | |
M. truncata | 0.001 | * | |
M. aequituberculata | 0.004 | * | |
A. latistella | 0.001 | * | |
A. digitifera | 0.001 | * | |
A. divaricata | 0.034 | * | |
Seawater | 0.001 | * | |
Note: The asterisk (*) indicates that the significance value is less than 0.05. |
Autumn | Winter | Spring | Summer | |
pH | 8.29 ± 0.16 | 8.12 ± 0.27 | 8.21 ± 0.24 | 8.07 ± 0.17 |
SST/℃ | 28.28 ± 1.13 | 24.03 ± 1.94 | 29.88 ± 1.36 | 30.63 ± 0.90 |
DO/mg·L−1 | 8.92 ± 2.92 | 7.48 ± 2.05 | 8.88 ± 2.22 | 7.75 ± 2.26 |
Nitrite/μg·L−1 | 3.72 ± 0.86 | 3.08 ± 0.54 | 1.79 ± 1.15 | 1.23 ± 0.22 |
Nitrate/μg·L−1 | 58.5 ± 5.07 | 26.5 ± 0.81 | 25.6 ± 5.17 | 23.3 ± 2.51 |
Ammonium/μg·L−1 | 30.3 ± 2.00 | 9.80 ± 0.77 | 35.5 ± 4.20 | 17.3 ± 1.35 |
Phosphate/μg·L−1 | 1.83 ± 1.44 | 0.286 ± 0.10 | 2.96 ± 2.42 | 4.48 ± 0.36 |