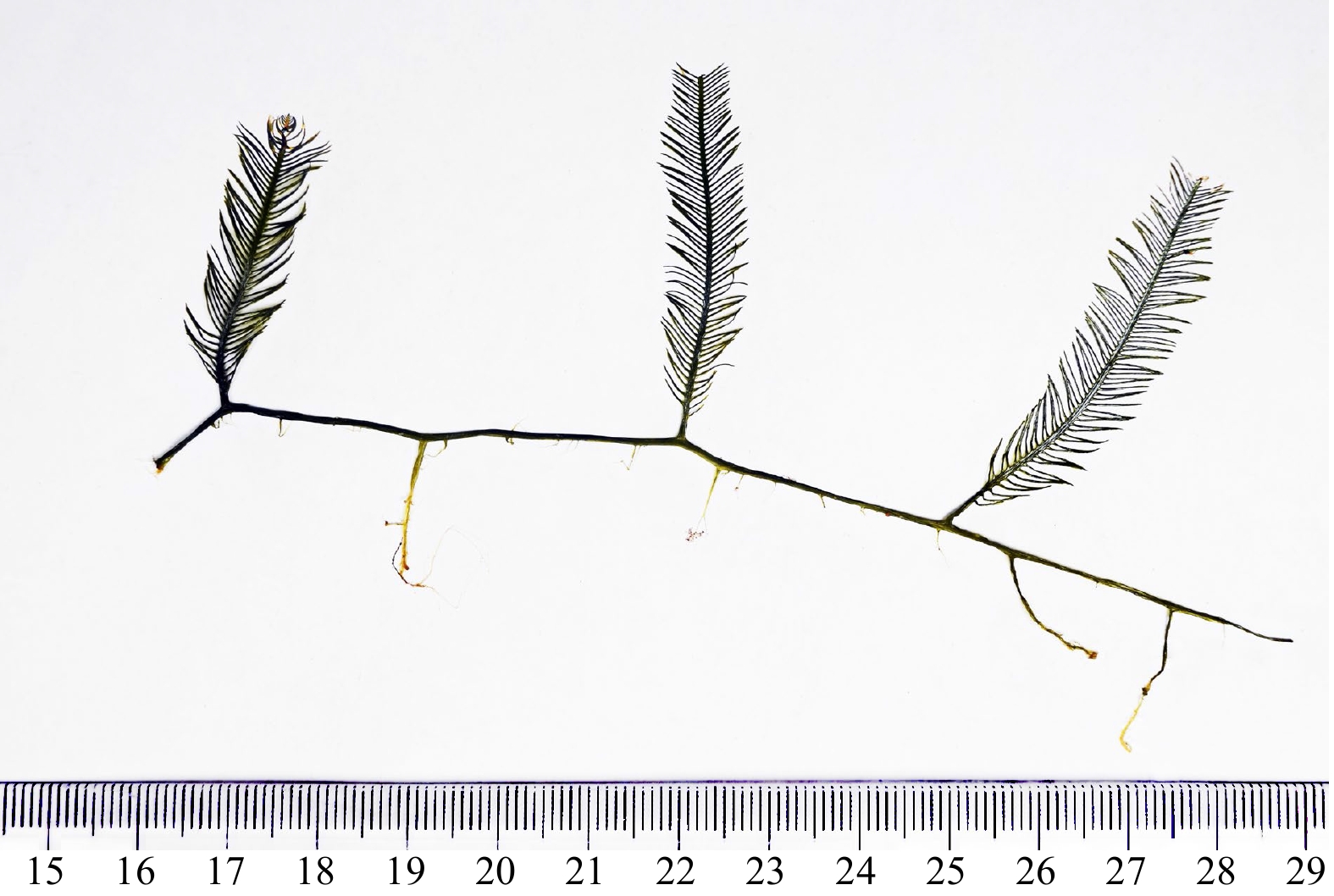
Citation: | Meng Liu, Fukang Qi, Yunpeng Lin, Yuping Yang, Jingping Xu. Influence of typhoon MITAG on the Kuroshio intrusion in the Luzon Strait during early fall 2019[J]. Acta Oceanologica Sinica, 2024, 43(9): 70-80. doi: 10.1007/s13131-024-2350-7 |
Caulerpa is a genus of marine green algae distributed mainly in tropical and subtropical waters and has more than 90 species. The thalli contain caulerpin, an alkaloid extracted from Caulerpa. The compound has anti-inflammatory activity, is rich in minerals and vitamins (Jiang et al., 2011), and has great value in application in the industries of food (de Gaillande et al., 2017), medicine (Gao, 2014; Chaves Filho et al., 2022), high-grade fertilizer (Wang, 2010), energy (Huang, 2012), and bioremediation of marine waters (Landi et al., 2022). Physiological and ecological studies have mostly focused on some environmental factors such as temperature (Li et al., 2022; Shi et al., 2022), salinity (Guo et al., 2015; Cai, 2021), irradiances (Stuthmann et al., 2021; Zhong et al., 2021), nutrient (Liu et al., 2016; Zhang et al., 2020) and heavy metal salt stress (Pang et al., 2021) for cultivated Caulerpa lentillifera in Southeast Asian countries. However, due to invasive nature, some species caused ecological problems in the Mediterranean, America, Australia, and other waters in the world (Davis et al., 1997; Meinesz et al., 2001; Verlaque et al., 2004; Anderson, 2005; Lapointe et al., 2005; Klein and Verlaque, 2008; Kang et al., 2021). In a eutrophic sea where some species boom excessively, vast and thick algal mats would form on the seafloor, which hamper sunlight transmission and seawater exchange, posing a large threat to local mariculture activities or coral reef growth (Williams and Schroeder, 2004). In environments with poor water quality, these blooming species have a strong ability to absorb nutrients from water and sediments (Williams, 1984; Kolar and Lodge, 2001). Therefore, they have the potential for sewage treatment (Landi et al., 2022).
Some of the Caulerpa species are highly invasive such as Caulerpa taxifolia and Caulerpa racemosa (Anderson, 2005; Fernández and Cortés, 2005; Liu et al., 2019). Caulerpa sertularioides (Anderson, 2005; Fernández and Cortés, 2005), also known as green feather alga, is similar in appearance and has the same mode of reproduction as C. taxifolia, including well-developed stolons and rhizoids for easy attachment to substrates (Fig. 1). It is highly invasive because its fragment of stolons can grow continuously from its apices (Smith and Walters, 1999), and spread quickly in the tropical northeast Pacific (Withgott, 2002). Its bloom damaged the coral reefs seriously (Smith et al., 2010). Some scientists believed that the high invasion ability might be related to a favorite temperature and the quick proliferation of thallus fragments (Fernández and Cortés, 2005). Some affected countries (such as Spain and France) are very concerned about the invasion and have tried to eradicate or control it by banning the trade of aquatic species (Klein and Verlaque, 2008).
In China, C. sertularioides was distributed mainly along the coast of the South China Sea in Taiwan, Hainan, and other islands (Ding et al., 2015; Liu et al., 2019). During the growing season, its blooms and the biomass abounds along the coast of the South China Sea (Fig. 2). However, few previous studies focused on its antimicrobial activities (Kumar et al., 2011), and polysaccharides and sterols (Shevchenko et al., 2009; Chaves Filho et al., 2022). More studies are required to elucidate its invasion characteristics and potential.
The response surface methodology (RSM) is a new statistical method for solving multivariable problems and exploring optimal process parameters by analyzing the regression equation (Mee, 2009; Mäkelä, 2017). It has been widely used in the research of life science in recent years because of its advantage of direct display selection of optimal operating conditions in experiment design (Kim et al., 2019; Srinivas et al., 2019; Faramarzi et al., 2019; da Silva et al., 2019; Vishwakarma and Banerjee, 2019; Ebadi et al., 2019; Nur et al., 2019). In China, the RSM method has recently been used to analyze the algal polysaccharides of Caulerpa lentillifera, demonstrating its advantages in the analysis of the active ingredients of algae (Tong et al., 2022).
In this study, effects of irradiance, temperature, and salinity on the growth of C. sertularioides are studied by using RSM. By determining and analyzing the optimum conditions of multiple ecological factors, it will provide reasonable data for cultivation, prevention and control of biological invasion of C. sertularioides in the natural waters.
The samples of C. sertularioides were bought from Tianjin’s aquariums and pre-cultured for one week in a seawater tank at room temperature, the thalli were washed and cleaned with disinfected seawater to remove debris, and then cultured under aeration for 30 days under the conditions of irradiance (37.5 ± 6.25) μmol/(m2·s), temperature (25 ± 1)℃, and salinity (30 ± 1) to obtain adequate biomass for the following experiment.
The thalli with long stolons were picked out and cut with sterilized scissors into at least 3 cm-long fragments carefully, making sure each fragment containing some blades and rhizoids (Smith and Walters, 1999), and placed in the air for 15 s for better wound healing. The fresh weight of each fragment was between 0.31–0.38 g (the mean standard deviation was (0.35 ± 0.02) g).
Referring to the cultivation experience and data, three ecological single-factor experiments, in which three main factors, e.g., irradiance, salinity, and temperature are tested and each is assigned a variable value, while the other two are constants. The conditions were set as: (1) irradiance at 18.75 μmol/(m2·s), 25.00 μmol/(m2·s), 31.25 μmol/(m2·s), 37.50 μmol/(m2·s), 43.75 μmol/(m2·s) and 50.00 μmol/(m2·s), salinity at 30 and temperature at 25℃; (2) temperature at 19℃, 22℃, 25℃, 28℃, 31℃ and 34℃, irradiance at 37.5 μmol/(m2·s) and salinity at 30; and (3) salinity at 15, 20, 25, 30, 35 and 40, irradiance at 37.5 μmol/(m2·s) and temperature at 25℃.
Each sample (including three fragments) was placed in a 250 mL conical flask containing 200 mL seawater that was renewed daily and cultured in the thermostatic illumination incubator (Jiangnan Instrument Co., Ltd., Ningbo, type GXZ) referring to the different treatments above. The total cultivation cycles last a week. Each treatment group was replicated three times. All samples were taken out and weighted wet at the end of the week, and then the SGR (specific growth rate) was calculated.
A central combination design experiment and the verification experiment on the results of the RSM of the three factors were performed using multi-factor experimental method. The results of the single-factor experiment were used to confirm the horizontal range of the ecological factor. The Box-Behnken central combination design, or the Box-Behnken design for simplicity—an RSM method was used to determine the SGR of the thalli fragments, and the optimum conditions of irradiance, temperature, and salinity.
The experimental data were statistically analyzed using Design-Expert 10.
SGR can be expressed as:
$$ {\rm{ SGR}} =\frac{W_t-W_0}{t} \times 100 \%, $$ | (1) |
where W0 is the fresh mass (g) of fragments at the initial stage; Wt is the fresh mass (g) of fragments at the end of the experiment; and t is the number of days of the experiment.
SGR of the fragments increased at first and then decreased with the increase of the variable factor. Peaks appeared at irradiance 37.5 μmol/(m2·s), temperature 25℃, and salinity 30, under which the SGR was 4.35%, 4.22%, and 4.33%, respectively (Figs 3–5).
Irradiance, temperature, and salinity are considered the three key factors affecting the growth of the species, and SGR is the response value. According to the results of the single-factor experiments, the horizontal ranges of the three factors were set and analyzed in the Box-Behnken design (Table 1), in which 17 three-factor combinations were determined and the SGRs of thalli fragments of the 17 combinations were measured (Table 2).
Factor | Irradiance /(μmol·m−2·s−1) | Temperature/℃ | Salinity |
Low | 31.25 | 22 | 25 |
High | 43.75 | 28 | 35 |
Serial number | Irradiance/ (μmol·m−2·s−1) | Temperature/℃ | Salinity | SGR/% |
1 | 37.5 | 22 | 35 | 2.84 |
2 | 37.5 | 25 | 30 | 4.62 |
3 | 37.5 | 28 | 35 | 3.06 |
4 | 37.5 | 25 | 30 | 4.52 |
5 | 37.5 | 22 | 25 | 2.46 |
6 | 43.75 | 28 | 30 | 3.25 |
7 | 31.25 | 22 | 30 | 1.93 |
8 | 37.5 | 25 | 30 | 4.52 |
9 | 43.75 | 22 | 30 | 3.20 |
10 | 31.25 | 28 | 30 | 2.65 |
11 | 43.75 | 25 | 25 | 3.41 |
12 | 37.5 | 25 | 30 | 4.70 |
13 | 31.25 | 25 | 35 | 2.63 |
14 | 37.5 | 28 | 25 | 3.26 |
15 | 31.25 | 25 | 25 | 2.38 |
16 | 43.75 | 25 | 35 | 3.38 |
17 | 37.5 | 25 | 30 | 4.62 |
Design-Expert 10 was used for the quadratic multiple regression of the data shown in Table 2. The regression equation was established as below:
$$ \begin{aligned}{\rm{SGR}}(\%)= &\;4.60+0.46A+0.22B+0.049C-0.17AB-0.074AC-\\&0.14BC-0.90A^2-0.94B^2-0.75C^2, \end{aligned} $$ | (2) |
where A is irradiance, B is temperature, and C is salinity. The influences of factors A and B were highly significant (P < 0.000 1), and those of factors AB and BC were extremely significant (P < 0.01).
In addition, as ANOVA results show, the model of regression equation was highly significant (P < 0.000 1), while the lack-of-fit was not significant (P > 0.05), thus the mode could better describe the real relationship between various factors and the response value (SGR) (Table 3). In our response surface analysis (Figs 6–11), the factors involved in multiple interactions were irradiance (in μmol/(m2·s)), temperature (℃), and SGR (%). The results show that within the set range, the SGR of the fragments increased first and then decreased with the increases in irradiance, temperature, and salinity, and the interactions of irradiance-temperature and temperature-salinity were extremely significant. According to the analysis of the model, the best combination condition for the growth of fragments was irradiance 39.03 μmol/(m2·s), temperature 25.29℃, and salinity 30.06, under which the SGR was the best, reaching 4.66%.
Source | Sum of squares | df | Mean square | F value | P value | Significance |
Model | 12.89 | 9 | 1.43 | 304.92 | <0.000 1 | Significant |
A−irradiance | 1.67 | 1 | 1.67 | 355.29 | <0.000 1 | − |
B−temperature | 0.4 | 1 | 0.4 | 85.16 | <0.000 1 | − |
C−salinity | 0.02 | 1 | 0.019 | 4.08 | 0.083 | − |
AB | 0.11 | 1 | 0.11 | 23.76 | 0.001 8 | − |
AC | 0.02 | 1 | 0.022 | 4.64 | 0.068 | − |
BC | 0.08 | 1 | 0.083 | 17.67 | 0.004 | |
A2 | 3.4 | 1 | 3.4 | 724.44 | <0.000 1 | − |
B2 | 3.74 | 1 | 3.74 | 796.58 | <0.000 1 | − |
C2 | 2.34 | 1 | 2.34 | 498.31 | <0.000 1 | − |
Residual | 0.033 | 7 | 4.70 × 10−3 | − | − | − |
Lack of fit | 9.07 × 10−3 | 3 | 3.02 × 10−3 | 0.51 | 0.80 | Insignificant |
Pure error | 0.024 | 4 | 5.95 × 10−3 | − | − | − |
Cor total | 12.93 | 16 | − | − | − | − |
Note: df: Degrees of Freedom. Cor total: This row shows the amount of variation around the mean of the observations. The model explains part of it, the residual explains the rest. |
To verify the optimum combination conditions, another experiment was conducted under the above-stated optimum condition. Within the scope of the precision of experimental instruments, the SGR of the thalli fragments was measured to be 4.66%, which is consistent overall with the predicted results of response surface method.
Some species of genus Caulerpa are common invasive green algae. Their growth and development are affected by local coastal environmental conditions. Taking C. racemosa as an example, its growth varied in temperature in terms of season, region, or water depth. As reported previously, its blade length reached 6 cm in October at 0–3 m depth in Leghorn, Italy (Piazzi and Cinelli, 1999), and at a deeper depth of 17 m in Marseille, France; its blade height in summer was on average 2 cm only and no such a summer peak was observed (Ruitton et al., 2005). Moreover, C. racemosa had fewer blades in winter at a depth of 2 m in coastal waters of northern Italy (Piazzi et al., 2001). In Japanese waters, Caulerpa species begin to develop in spring and become mature in summer (Wang, 2015). In salinity and irradiance, C. racemosa grew fastest in salinity 30–40 and light intensity 20–60 μE/(m2·s) as reported in the intertidal zone/subtidal reef of southwestern coastal Australia (Carruthers et al., 1993). In China, a study showed that the optimum conditions for C. sertularioides growth were 26℃, salinity 27.5, and irradiance 25 μmol/(m2·s) (Zhong et al., 2021). Similarly, C. racemosa on Taiwan Island in China grew best in seawater temperatures ranging from 24–28℃, while the biomass reduced dramatically below 22℃ or above 31℃ (Shi, 2008).
All these data provide references for the monitoring and control of the invasion of Caulerpa species. However, the above-mentioned cases are complicated and imprecise among the three parameters. Therefore, RSM was introduced and applied to this study.
RSM is a commonly used method for experimental design, which is applicable for multi-factor and multi-level experimental designs and is convenient, and has good predictability (Stensrud et al., 2000; Nazzal et al., 2002; Kramar et al., 2003; Hadiyat et al., 2022). Currently, it is widely used for biological enzyme medium configuration and in food processing (Zhao et al., 2013; Gong et al., 2022; Pinheiro et al., 2022). In this study, we first determined preliminarily growth conditions of C. sertularioides fragments in a single-factor manner: 25℃ in temperature, 30 in salinity, and 37.5 μmol/(m2·s) in irradiance, under which the SGR was the best. Subsequently, the interactions among irradiance, temperature, and salinity, and an optimum ecological multi-factor combination condition were established and analyzed in RSM.
The results of RSM show that the interactions between irradiance and temperature, and temperature and salinity were extremely significant. Temperature regulates algal growth by affecting enzyme activity (Wang et al., 2014; Feng et al., 2021). Salinity regulates ion exchange by affecting osmotic pressure (Flexas et al., 2004). The enzymes require the activation of specific ions (Wells and Di Cera, 1992); too high or too low salinity could affect the activity of enzymes or their carriers (Okur et al., 2002). The irradiance mainly affects the photosynthesis of algae (Dennison, 1987), in which certain enzymes are involved (Bischof et al., 2000). The interactions between temperature and salinity, and between temperature and irradiance have been observed to be significant in other algae Prorocentrum donghaiense (Xu et al., 2010) and Skeletonema costatum (Yu, 2005).
The optimum combination condition indicated by RSM was: irradiance 39.03 μmol/(m2·s), temperature 25.29℃, and salinity 30.06. The R2Adj (adjusted coefficient of determination) of the multiple correlation coefficient R after the analysis of variance was 0.99, indicating that 99% of the change in the response value is derived from the selected variable, which means that the error of this experiment is very small. By analyzing the response surface of the interaction terms in the regression equation (Figs 9–11), we found that the interaction among temperature, salinity, and irradiance is significant in the selected range, which is consistent with the result of the model analysis (Table 3), indicating that the model could be used to optimize the growth conditions of the fragments of C. sertularioides, and to predict its SGR. The SGR value determined by the verification experiment was higher than the maximum SGR of the single-factor experiment, and also higher than the SGR of C. sertularioides measured under 16 of the total 17 combined conditions determined in the Box-Behnken design (see Table 2), but slightly lower than one of the conditions, which is speculated that it was caused by an experimental error. The result indicates that the optimum combination conditions of ecological factors for the growth of C. sertularioides optimized by RSM (irradiance 39.03 μmol/(m2·s), temperature 25.29℃, and salinity 30.06) are suitable for the growth of C. sertularioides. In addition, it also indicates that the optimum combination conditions (the irradiance, temperature, and salinity) of ecological factors for the growth of C. sertularioides optimized in this study can be taken as the center and be appropriately extended to combine in the range of appropriate growth conditions, which provided new theoretical data and solutions for the cultivation, invasion prediction, and monitoring of Caulerpa species in China and around the world, and offer some new scientific data for future in-depth researches in this regard. Based on the literatures and our result, with further research and data mining, we predict that the RSM method will be better applied in the following aspects of macroalgae: (1) species or taxa (new cultivars) that have not been studied because an optimal set of culture conditions need to be obtained; (2) germplasms that require intensive orientation cultured, where changes in the microenvironment often cause them to undergo qualitative changes, such as the transition from the growth to the reproductive stage; (3) the analysis of environmental hazards of cultivated species in the field, which facilitates the acquisition of new insights.
As known from the current works of literature, those blooming macroalgae generally adapt to their environment very quickly through multiple pathways, which means they are extremely viable. C. sertularioides is also extremely adaptable to its environment which is similar to other Caulerpa species. In addition to sexual reproduction (which had few been seen in the literature), it can grow and spread on the seafloor through its stolons and fragments or branches. In them, the rate of speed by the fragments is much faster. In the previous research and cultivations of Caulerpa species, asexual materials were generally used, mainly fragments or branches. They are much more economical and conveniently available than sexual ones. Therefore, our experiment was implemented using fragments rather than whole individuals.
The effects of ecological factors on growth of C. sertularioides, an invasive potential blooming green alga, were studied. Its optimum conditions of irradiance, temperature and salinity for the growth of its fragments were determined in the response surface methodology (RSM). Using the Box-Behnken design, the conditions were optimized and verified to be irradiance 39.03 μmol/(m2·s), temperature 25.29℃, and salinity 30.06, under which the SGR reached 4.66%. As the research progresses and the data are fully explored, the RSM method may have great potential application in the environmental adaptation characteristics of new macroalgal cultivars, intensive orientation cultured germplasms, and environmental hazard analysis of cultivated species in the field.
We thank Roger Z. YU, a Canadian english editor for help in modifying the language.
Caruso M J, Gawarkiewicz G G, Beardsley R C. 2006. Interannual variability of the Kuroshio intrusion in the South China Sea. Journal of Oceanography, 62(4): 559–575, doi: 10.1007/s10872-006-0076-0
|
Centurioni L R, Niiler P P, Lee D K. 2004. Observations of inflow of philippine sea surface water into the South China Sea through the luzon strait. Journal of Physical Oceanography, 34(1): 113–121, doi: 10.1175/1520-0485(2004)034<0113:OOIOPS>2.0.CO;2
|
Chang Yu-Chia, Tseng Ruo-Shan, Centurioni L R. 2010. Typhoon-induced strong surface flows in the Taiwan Strait and Pacific. Journal of Oceanography, 66(2): 175–182, doi: 10.1007/s10872-010-0015-y
|
Chen Fei, Du Yan, Yan Li, et al. 2010. Response of upper ocean currents to typhoons at two ADCP moorings west of the Luzon Strait. Chinese Journal of Oceanology and Limnology, 28(5): 1002–1011, doi: 10.1007/s00343-010-0025-z
|
de Boyer Montégut C, Madec G, Fischer A S, et al. 2004. Mixed layer depth over the global ocean: An examination of profile data and a profile-based climatology. Journal of Geophysical Research: Oceans, 109(C12): C12003
|
Gao Shumin, Han Shuzong, Wang Shicheng, et al. 2022. The influence of typhoon ‘Hongxia’ on the intrusion of the Kuroshio current into the South China Sea. Journal of Ocean University of China, 22(2): 297–312
|
Garau B, Ruiz S, Zhang W G, et al. 2011. Thermal lag correction on slocum CTD glider data. Journal of Atmospheric and Oceanic Technology, 28(9): 1065–1071, doi: 10.1175/JTECH-D-10-05030.1
|
Guo Lin, Xiu Peng, Chai Fei, et al. 2017. Enhanced chlorophyll concentrations induced by Kuroshio intrusion fronts in the northern South China Sea. Geophysical Research Letters, 44(22): 11565–11572
|
He Yihao, Lin Xiayan, Han Guoqing, et al. 2024. The different dynamic influences of typhoon kalmaegi on two pre-existing anticyclonic ocean eddies. Ocean Science, 20(2): 621–637, doi: 10.5194/os-20-621-2024
|
Hsin Yi-Chia, Qu Tangdong, Wu Chau-Ron. 2010. Intra-seasonal variation of the Kuroshio southeast of Taiwan and its possible forcing mechanism. Ocean Dynamics, 60(5): 1293–1306, doi: 10.1007/s10236-010-0294-2
|
Hsu Taiwen, Chou Meng-Hsien, Chao Weiting, et al. 2018. Typhoon effect on Kuroshio and green island wakes: A modelling study. Atmosphere, 9(2): 36, doi: 10.3390/atmos9020036
|
Hu Jianyu, Zheng Quanan, Sun Zhenyu, et al. 2012. Penetration of nonlinear Rossby eddies into South China Sea evidenced by cruise data. Journal of Geophysical Research: Oceans, 117(C3): C03010
|
Huang Zhida, Hu Jianyu. 2010. Hydrographical characteristic along the 20.5°N section through the Luzon Strait based on Argo data. Journal of Oceanography in Taiwan Strait (in Chinese), 29(4): 539–546
|
Idronaut S R L, 2019. OCEAN SEVEN 304Plus CTD PROBE. Brugherio: Monte Amiata, 9–10
|
Kuo Yichun, Zheng Zhewen, Zheng Quanan, et al. 2018. Typhoon-Kuroshio interaction in an air-sea coupled system: Case study of typhoon nanmadol (2011). Ocean Modelling, 132: 130–138, doi: 10.1016/j.ocemod.2018.10.007
|
Li Shufeng, Wang Shuxin, Zhang Fumin, et al. 2019. Constructing the three-dimensional structure of an anticyclonic eddy in the south China sea using multiple underwater gliders. Journal of Atmospheric and Oceanic Technology, 36(12): 2449–2470, doi: 10.1175/JTECH-D-19-0006.1
|
Li Shufeng, Zhang Fumin, Wang Shuxin, et al. 2020. Constructing the three-dimensional structure of an anticyclonic eddy with the optimal configuration of an underwater glider network. Applied Ocean Research, 95: 101893, doi: 10.1016/j.apor.2019.101893
|
Liang Wen-Der, Yang Yiing Jang, Tang Tswen Yung, et al. 2008. Kuroshio in the Luzon Strait. Journal of Geophysical Research: Oceans, 113(C8): C08048
|
Lien R C, Ma B, Cheng Yu-Hsin, et al. 2014. Modulation of Kuroshio transport by mesoscale eddies at the Luzon Strait entrance. Journal of Geophysical Research: Oceans, 119(4): 2129–2142, doi: 10.1002/2013JC009548
|
Liu Guangping, Hu Jianyu. 2012. A preliminary analysis of variation of the Kuroshio axis during tropical cyclone. Journal of Tropical Oceanography (in Chinese), 31(1): 35–41
|
Liu Yupeng, Tang Danling, Tang Shilin, et al. 2020. A case study of Chlorophyll a response to tropical cyclone Wind Pump considering Kuroshio invasion and air-sea heat exchange. Science of The Total Environment, 741: 140290, doi: 10.1016/j.scitotenv.2020.140290
|
Liu Meng, Wang Zhiwen, Yu Kaiqi, et al. 2023. Two distinct types of turbidity currents observed in the Manila Trench, South China Sea. Communications Earth & Environment, 4: 108
|
Liu Yonggang, Weisberg R H, Lembke C. 2015. Glider salinity correction for unpumped CTD sensors across a sharp thermocline. In: Liu Yonggang, Kerkering H, Weisberg R H, eds. Coastal Ocean Observing Systems. Boston: Academic Press, 305–325.
|
Lu Jiuyou, Liu Qinyu. 2013. Gap-leaping Kuroshio and blocking westward-propagating Rossby wave and eddy in the Luzon Strait. Journal of Geophysical Research: Oceans, 118(3): 1170–1181, doi: 10.1002/jgrc.20116
|
Ma Jie, Hu Shijian, Hu Dunxin, et al. 2022. Structure and variability of the Kuroshio and Luzon Undercurrent observed by a mooring array. Journal of Geophysical Research: Oceans, 127(2): e2021JC017754, doi: 10.1029/2021JC017754
|
Ma Wei, Wang Yanhui, Yang Shaoqiong, et al. 2018. Observation of internal solitary waves using an underwater glider in the northern South China Sea. Journal of Coastal Research, 34(5): 1188–1195
|
Morison J, Andersen R, Larson N, et al. 1994. The correction for thermal-lag effects in Sea-Bird CTD data. Journal of Atmospheric and Oceanic Technology, 11(4): 1151–1164, doi: 10.1175/1520-0426(1994)011<1151:TCFTLE>2.0.CO;2
|
Nan Feng, Xue Huijie, Chai Fei, et al. 2011. Identification of different types of Kuroshio intrusion into the South China Sea. Ocean Dynamics, 61(9): 1291–1304, doi: 10.1007/s10236-011-0426-3
|
Nan Feng, Xue Huijie, Yu Fei. 2015. Kuroshio intrusion into the South China Sea: A review. Progress in Oceanography, 137: 314–333, doi: 10.1016/j.pocean.2014.05.012
|
Qian Simeng, Wei Hao, Xiao Jingen, et al. 2018. Impacts of the Kuroshio intrusion on the two eddies in the northern South China Sea in late spring 2016. Ocean Dynamics, 68(12): 1695–1709, doi: 10.1007/s10236-018-1224-y
|
Qu Tangdong. 2000. Upper-layer circulation in the South China Sea. Journal of Physical Oceanography, 30(6): 1450–1460, doi: 10.1175/1520-0485(2000)030<1450:ULCITS>2.0.CO;2
|
Qu Tangdong. 2002. Evidence for water exchange between the South China Sea and the Pacific Ocean through the Luzon Strait. Acta Oceanologica Sinica, 21(2): 175–185
|
Sui Junpeng, Chen Haijun, Wang Zhuyu, et al. 2018. Analysis of the Luzon Strait transport anomalies caused by typhoon “Dandelion” in 2004. Marine Forecasts (in Chinese), 35(5): 1–6
|
Sun Jingru, Oey L, Xu F H, et al. 2017. Sea level rise, surface warming, and the weakened buffering ability of South China Sea to strong typhoons in recent decades. Scientific Reports, 7(1): 7418, doi: 10.1038/s41598-017-07572-3
|
Sun Liang, Yang Yuanjian, Fu Yunfei. 2009. Impacts of typhoons on the Kuroshio large meander: Observation evidences. Atmospheric and Oceanic Science Letters, 2(1): 45–50, doi: 10.1080/16742834.2009.11446772
|
Tian Jiwei, Yang Qingxuan, Liang Xinfeng, et al. 2006. Observation of Luzon Strait transport. Geophysical Research Letters, 33(19): L19607
|
Troupin C, Beltran J P, Heslop E, et al. 2015. A toolbox for glider data processing and management. Methods in Oceanography, 13–14: 13–23
|
Wang Xiangpeng, Du Yan, Zhang Yuhong, et al. 2021. Influence of two eddy pairs on high-salinity water intrusion in the northern South China Sea during fall-winter 2015/2016. Journal of Geophysical Research: Oceans, 126(6): e2020JC016733, doi: 10.1029/2020JC016733
|
Wang Xiangpeng, Du Yan, Zhang Yuhong, et al. 2023. Effects of multiple dynamic processes on chlorophyll variation in the Luzon Strait in summer 2019 based on glider observation. Journal of Oceanology and Limnology, 41(2): 469–481, doi: 10.1007/s00343-022-1416-7
|
Wang Yanhui, Zhang Yiteng, Zhang Mingming, et al. 2017. Design and flight performance of hybrid underwater glider with controllable wings. International Journal of Advanced Robotic Systems, 14(3): 1729881417703566
|
Wu Chau-Ron, Hsin Y C. 2012. The forcing mechanism leading to the Kuroshio intrusion into the South China Sea. Journal of Geophysical Research: Oceans, 117(C7): C07015
|
Xue Huijie, Chai Fei, Pettigrew N, et al. 2004. Kuroshio intrusion and the circulation in the South China Sea. Journal of Geophysical Research: Oceans, 109(C2): C02017
|
Yang Yikai, Wang Dongxiao, Wang Qiang, et al. 2019. Eddy-induced transport of saline Kuroshio water into the northern South China Sea. Journal of Geophysical Research: Oceans, 124(9): 6673–6687, doi: 10.1029/2018JC014847
|
Yaremchuk M, Qu Tangdong. 2004. Seasonal variability of the large-scale currents near the coast of the Philippines. Journal of Physical Oceanography, 34(4): 844–855, doi: 10.1175/1520-0485(2004)034<0844:SVOTLC>2.0.CO;2
|
Yi Zhenhui, Yu Jiancheng, Mao Huabin, et al. 2019. A noise processing method for salinity data underwater glider. Journal of Unmanned Undersea Systems (in Chinese), 27(5): 503–513
|
Yuan Dongliang, Han Weiqing, Hu Dunxin. 2006. Surface Kuroshio path in the Luzon Strait area derived from satellite remote sensing data. Journal of Geophysical Research: Oceans, 111(C11): C11007
|
Zhang Zhiwei, Zhao Wei, Tian Jiwei, et al. 2015. Spatial structure and temporal variability of the zonal flow in the Luzon Strait. Journal of Geophysical Research: Oceans, 120(2): 759–776, doi: 10.1002/2014JC010308
|
Zhang Zheliang, Zheng Yunxia, Li Hao. 2023. Imprints of tropical cyclone on three-dimensional structural characteristics of mesoscale oceanic eddies. Frontiers in Earth Science, 10: 1057798, doi: 10.3389/feart.2022.1057798
|
Zhou Hui, Nan Feng, Shi Maochong, et al. 2009. Characteristics of water exchange in the Luzon Strait during September 2006. Chinese Journal of Oceanology and Limnology, 27(30): 650–657
|
Zhou Hui, Yang Wenlong, Liu Hengchang, et al. 2017. The influence of typhoon Haima on warm eddies near the Luzon Strait and its dynamics. Oceanologia et Limnologia Sinica (in Chinese), 48(6): 1276–1288
|
Factor | Irradiance /(μmol·m−2·s−1) | Temperature/℃ | Salinity |
Low | 31.25 | 22 | 25 |
High | 43.75 | 28 | 35 |
Serial number | Irradiance/ (μmol·m−2·s−1) | Temperature/℃ | Salinity | SGR/% |
1 | 37.5 | 22 | 35 | 2.84 |
2 | 37.5 | 25 | 30 | 4.62 |
3 | 37.5 | 28 | 35 | 3.06 |
4 | 37.5 | 25 | 30 | 4.52 |
5 | 37.5 | 22 | 25 | 2.46 |
6 | 43.75 | 28 | 30 | 3.25 |
7 | 31.25 | 22 | 30 | 1.93 |
8 | 37.5 | 25 | 30 | 4.52 |
9 | 43.75 | 22 | 30 | 3.20 |
10 | 31.25 | 28 | 30 | 2.65 |
11 | 43.75 | 25 | 25 | 3.41 |
12 | 37.5 | 25 | 30 | 4.70 |
13 | 31.25 | 25 | 35 | 2.63 |
14 | 37.5 | 28 | 25 | 3.26 |
15 | 31.25 | 25 | 25 | 2.38 |
16 | 43.75 | 25 | 35 | 3.38 |
17 | 37.5 | 25 | 30 | 4.62 |
Source | Sum of squares | df | Mean square | F value | P value | Significance |
Model | 12.89 | 9 | 1.43 | 304.92 | <0.000 1 | Significant |
A−irradiance | 1.67 | 1 | 1.67 | 355.29 | <0.000 1 | − |
B−temperature | 0.4 | 1 | 0.4 | 85.16 | <0.000 1 | − |
C−salinity | 0.02 | 1 | 0.019 | 4.08 | 0.083 | − |
AB | 0.11 | 1 | 0.11 | 23.76 | 0.001 8 | − |
AC | 0.02 | 1 | 0.022 | 4.64 | 0.068 | − |
BC | 0.08 | 1 | 0.083 | 17.67 | 0.004 | |
A2 | 3.4 | 1 | 3.4 | 724.44 | <0.000 1 | − |
B2 | 3.74 | 1 | 3.74 | 796.58 | <0.000 1 | − |
C2 | 2.34 | 1 | 2.34 | 498.31 | <0.000 1 | − |
Residual | 0.033 | 7 | 4.70 × 10−3 | − | − | − |
Lack of fit | 9.07 × 10−3 | 3 | 3.02 × 10−3 | 0.51 | 0.80 | Insignificant |
Pure error | 0.024 | 4 | 5.95 × 10−3 | − | − | − |
Cor total | 12.93 | 16 | − | − | − | − |
Note: df: Degrees of Freedom. Cor total: This row shows the amount of variation around the mean of the observations. The model explains part of it, the residual explains the rest. |
Factor | Irradiance /(μmol·m−2·s−1) | Temperature/℃ | Salinity |
Low | 31.25 | 22 | 25 |
High | 43.75 | 28 | 35 |
Serial number | Irradiance/ (μmol·m−2·s−1) | Temperature/℃ | Salinity | SGR/% |
1 | 37.5 | 22 | 35 | 2.84 |
2 | 37.5 | 25 | 30 | 4.62 |
3 | 37.5 | 28 | 35 | 3.06 |
4 | 37.5 | 25 | 30 | 4.52 |
5 | 37.5 | 22 | 25 | 2.46 |
6 | 43.75 | 28 | 30 | 3.25 |
7 | 31.25 | 22 | 30 | 1.93 |
8 | 37.5 | 25 | 30 | 4.52 |
9 | 43.75 | 22 | 30 | 3.20 |
10 | 31.25 | 28 | 30 | 2.65 |
11 | 43.75 | 25 | 25 | 3.41 |
12 | 37.5 | 25 | 30 | 4.70 |
13 | 31.25 | 25 | 35 | 2.63 |
14 | 37.5 | 28 | 25 | 3.26 |
15 | 31.25 | 25 | 25 | 2.38 |
16 | 43.75 | 25 | 35 | 3.38 |
17 | 37.5 | 25 | 30 | 4.62 |
Source | Sum of squares | df | Mean square | F value | P value | Significance |
Model | 12.89 | 9 | 1.43 | 304.92 | <0.000 1 | Significant |
A−irradiance | 1.67 | 1 | 1.67 | 355.29 | <0.000 1 | − |
B−temperature | 0.4 | 1 | 0.4 | 85.16 | <0.000 1 | − |
C−salinity | 0.02 | 1 | 0.019 | 4.08 | 0.083 | − |
AB | 0.11 | 1 | 0.11 | 23.76 | 0.001 8 | − |
AC | 0.02 | 1 | 0.022 | 4.64 | 0.068 | − |
BC | 0.08 | 1 | 0.083 | 17.67 | 0.004 | |
A2 | 3.4 | 1 | 3.4 | 724.44 | <0.000 1 | − |
B2 | 3.74 | 1 | 3.74 | 796.58 | <0.000 1 | − |
C2 | 2.34 | 1 | 2.34 | 498.31 | <0.000 1 | − |
Residual | 0.033 | 7 | 4.70 × 10−3 | − | − | − |
Lack of fit | 9.07 × 10−3 | 3 | 3.02 × 10−3 | 0.51 | 0.80 | Insignificant |
Pure error | 0.024 | 4 | 5.95 × 10−3 | − | − | − |
Cor total | 12.93 | 16 | − | − | − | − |
Note: df: Degrees of Freedom. Cor total: This row shows the amount of variation around the mean of the observations. The model explains part of it, the residual explains the rest. |